Guidance on waterborne pathogens in drinking water
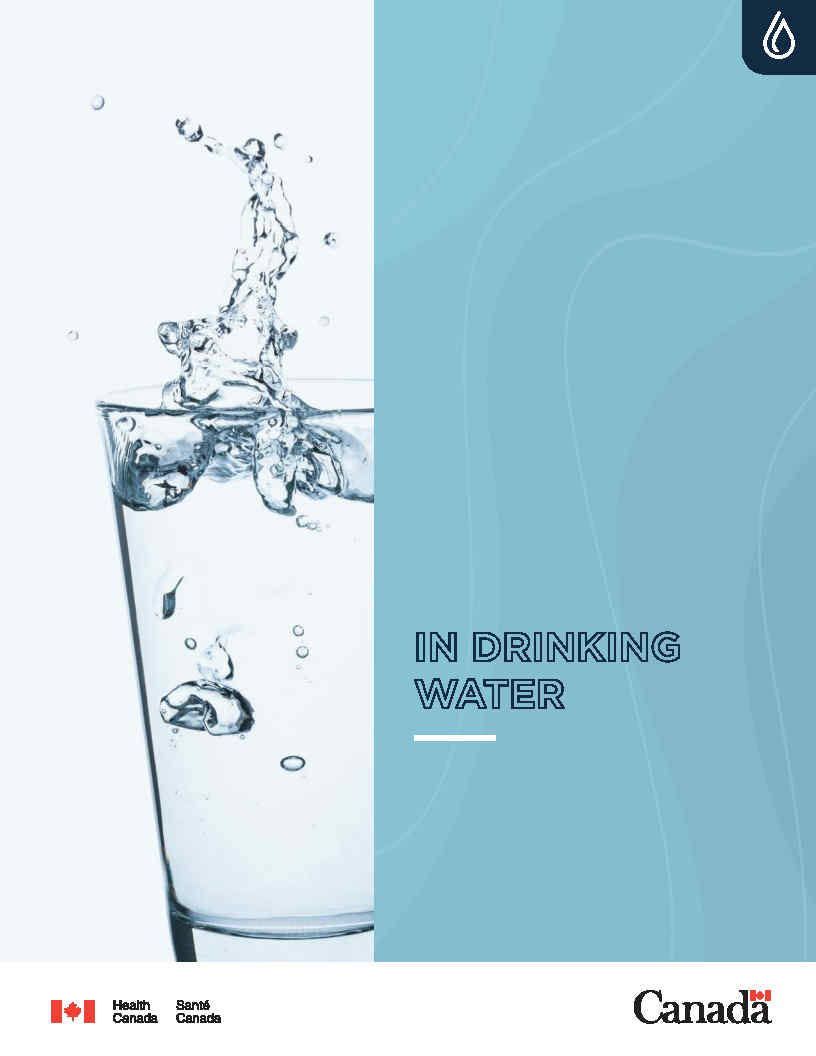
Download the alternative format
(PDF format, 1.29 MB, 100 pages)
Organization: Health Canada
Published: September 2022
Background on guidance documents
Health Canada works with the provinces, territories and federal agencies to establish the Guidelines for Canadian Drinking Water Quality. Over the years, new methodologies and approaches have led Health Canada, in collaboration with the Federal-Provincial-Territorial Committee on Drinking Water, to develop a new type of document, guidance documents, to provide advice and guidance on issues related to drinking water quality for parameters that do not require a formal Guideline for Canadian Drinking Water Quality.
Guidance documents are developed to provide operational or management guidance related to specific drinking water–related issues (e.g., boil water advisories) in order to make health risk assessment information available when a guideline value is not deemed necessary.
Guidelines are established under the Guidelines for Canadian Drinking Water Quality specifically for contaminants that meet all of the following criteria:
- exposure to the contaminant could lead to adverse health effects;
- the contaminant is frequently detected or could be expected to be found in a large number of drinking water supplies throughout Canada; and
- the contaminant is detected, or could be expected to be detected, at a level that is of possible health significance.
If a contaminant of interest does not meet all these criteria, Health Canada, in collaboration with the Federal-Provincial Territorial Committee on Drinking Water, may choose not to develop a Guideline Technical Document. In that case, a guidance document may be developed.
Guidance documents undergo a similar process as Guideline Technical Documents, including public consultations through the Health Canada Web site. They are offered as information for drinking water authorities and to help provide guidance in spill or other emergency situations.
Part A of this document provides guidance for managing risks associated with the pathogens discussed in this document; Part B provides scientific and technical information to support this guidance; Part C provides the references; and Part D provides the appendices.
Executive summary
Many types of pathogenic microorganisms can spread through drinking water, which may lead to human illness. Some are present in human or animal feces and can cause gastrointestinal illness when fecally-contaminated water is consumed. Others are naturally found in aquatic environments and can cause opportunistic infections when the conditions in engineered water systems (e.g., drinking water distribution systems and building/premise plumbing) allow them to multiply and spread primarily to individuals who are susceptible to infection, such as infants, the elderly and immunocompromised individuals. The health effects caused by these opportunistic pathogens are diverse and range from respiratory illness to infections of the eye, skin, central nervous system or the gastrointestinal tract. In severe cases, illnesses caused by opportunistic pathogens can be fatal.
A basic understanding of the different types of waterborne pathogens, their sources, the measures that are important for their control and the people that are most at risk for becoming sick is necessary for effective drinking water management and for preventing waterborne disease. This guidance document was prepared in collaboration with the Federal-Provincial-Territorial Committee on Drinking Water and describes these microorganisms, their health effects, how they are transmitted and best practices to ensure safe drinking water.
Assessment
Setting maximum acceptable concentrations for the pathogens described in this document remains impractical and is not necessary in order for drinking water utilities to adequately manage risks. Implementing a source-to-tap risk management approach is a universally recommended strategy for minimizing the growth and transmission of waterborne pathogens in drinking water and controlling their potential risks. Important elements of this strategy include source water protection, treatment requirements based on health-based treatment goals for enteric protozoa (Giardia and Cryptosporidium) and enteric viruses, and minimizing microorganism survival and growth in drinking water distribution systems. Maintaining microbiological control in water systems in buildings and residences is also a critical component of providing safe drinking water at the consumer’s tap.
The intent of this document is to provide stakeholders, such as provincial and territorial regulatory authorities, decision makers, water system owners and operators, building owners and managers, and consultants with guidance on waterborne pathogens in drinking water that are not addressed in the Guidelines for Canadian Drinking Water Quality, with the objective of minimizing public health risks through Canadian water systems.
Table of contents
- Part A
- Part B. Technical information
- B.1 Bacteria of gastrointestinal origin
- B.2 Naturally-occurring pathogens
- Part C. References
- Part D: Appendices
- Appendix A: List of Abbreviations
- Appendix B: Table B1 - Summary of waterborne pathogens of gastrointestinal origin
- Appendix C: Table C1 - Summary of waterborne naturally-occurring pathogens
- Appendix D: Figure D1 - Relative CT values for various waterborne pathogens, free chlorine (2 log inactivation, 5-25°C, pH 6-9)
- Appendix E: Figure E1 - Relative UV dose requirements for various waterborne pathogens (4 log inactivation)
Part A
A.1 Goal and scope
The goal of this document is to provide provinces, territories, other government departments and stakeholders with guidance on waterborne pathogens of potential human health concern that are not addressed elsewhere in the Guidelines for Canadian Drinking Water Quality.
A wealth of important research has advanced understanding of the public health relevance of these waterborne pathogens in drinking water systems. Management strategy considerations focus on the treatment plant and the distribution system; however, some guidance is provided on source water protection and for plumbing systems in buildings and residences.
A.2 Introduction
The microorganisms covered in this document are listed in Table 1. This document addresses the waterborne bacterial pathogens that are of gastrointestinal origin and are known to cause illness when there is fecal contamination of inadequately treated drinking water. The document also describes naturally-occurring waterborne pathogens, as these microorganisms are often associated with infections, and in extreme cases, deaths in susceptible individuals (such as infants, the elderly and immunocompromised individuals), and are referred to as opportunistic pathogens. Engineered water systems (e.g., drinking water distribution systems and building/premise plumbing) are important habitats for naturally-occurring waterborne pathogens. Many possess features that present challenges for drinking water utilities and building owners/managers, such as increased resistance to disinfection, growth under low nutrient and oxygen conditions and growth in biofilms. Effective management of these microorganisms requires collaboration between water utilities that manage drinking water distribution systems and those responsible for building plumbing systems.
Overall strategies for management are summarized in Part A.3. In Part B, brief technical information is presented on the individual pathogens (See Table 1), their effects on human health, as well as sources and exposure. Analytical and treatment considerations are also summarized.
Waterborne pathogens of gastrointestinal origin
Campylobacter spp.
Enteric pathogenic Escherichia coli(E. coli) and Shigella spp.
Helicobacter pylori
Salmonella spp.
Yersinia spp.
Waterborne naturally-occurring pathogens
Bacteria:
Aeromonas spp.
Legionella spp.
Mycobacterium spp.
Pseudomonas spp.
Protozoa:
Acanthamoeba spp.
Naegleria fowleri
A.3 Management strategies
Setting maximum acceptable concentrations for these microorganisms remains impractical and is not required in order for drinking water utilities and building owners/managers to adequately manage risks. Instead, a priority focus on drinking water process management, for example, through the implementation of a source-to-tap or water safety plan approach, is the recommended strategy for water utilities to manage potential risks. Water utilities should also have education and outreach programs to make consumers aware of how water quality can deteriorate within residential and building plumbing systems. Building water management plans are the recommended best practice for building owners/managers.
It is important that those responsible for managing drinking water quality be aware that the absence of indicator bacteria (e.g., Escherichia coli, total coliforms) does not indicate the absence of opportunistic pathogens such as Legionella and Mycobacterium. The latter are more resistant to disinfection. As a result, water utilities and building owners/managers should have appropriate risk management strategies that utilize multiple parameters to assess the performance of drinking water treatment and/or distribution operations.
A.3.1 Source water protection
Source water protection is the first step in a source-to-tap, or water safety plan approach. Source water assessments should include: the identification of potential sources of microbiological contamination in the watershed/aquifer; potential pathways and/or events (low to high risk) by which microorganisms of concern can make their way into the source water and affect water quality; and conditions likely to lead to peak concentrations. Assessments for subsurface sources should include, at a minimum, a hydrogeological assessment, an evaluation of well integrity and a survey of activities and physical features in the area (Health Canada 2019b, 2019c). It is important that risks from both land-based and aerosol-generating activities (e.g., spray irrigation, pressure washers) be considered in the source water assessment.
Nutrients are critical for microorganisms to survive, grow and spread. Thus, surface and subsurface sources should be characterized with regard to organic and inorganic nutrient concentrations (Cantor, 2017).
Water temperature may also be an important consideration (Health Canada, 2021). Enteric bacteria (see Section B.1) survive longer at low temperatures whereas opportunistic pathogens (see Section B.2) thrive at warmer temperatures.
A.3.2 Water treatment plant
When properly designed and operated, physical removal and disinfection technologies commonly used in drinking water treatment are expected to control the risks associated with the microorganisms discussed in this guidance document. However, it is important to recognize that treated drinking water is not sterile and naturally-occurring bacterial pathogens (see Section B.2.1) have been detected in treated water. Adverse health effects from these opportunistic pathogens are not expected at the concentrations measured in treated water (Le Dantec et al., 2002) but these microorganisms can spread to and grow in downstream biofilms (Brooks et al., 2004). Water utilities should therefore aim to produce biologically stable water to minimize the potential for problems to occur in distribution and premise plumbing systems (see Sections A.3.3 and A.3.4) (Health Canada, 2022). Important elements related to drinking water treatment include:
- optimize treatment performance for turbidity and natural organic matter removal;
- properly apply primary disinfection technologies to meet appropriate CT (disinfectant concentration in mg/L × time in minutes) requirements when using chemical oxidants (i.e., free chlorine, ozone, chlorine dioxide) or IT (intensity measured in mW/cm2 or W/m 2× time measured in seconds resulting in a computed fluence in mJ/cm2) requirements when using ultraviolet (UV) disinfection;
- minimize nutrient concentrations in treated water and have a good understanding of their concentrations in the distribution system;
- optimize treatment to minimize the amount of scaling and/or corrosion in the distribution system;
- properly apply secondary disinfection technologies (i.e., free chlorine or monochloramine) for residual maintenance in the distribution system;
- conduct performance testing using multiple parameters (e.g., disinfectant residual, microbiological indicators, pH, turbidity);
- provide operator training to assure the effectiveness of the water safety plan at all times.
It is important to note that monochloramine is recommended only for secondary disinfection and residual maintenance in the distribution system (Health Canada, 2019b).
A.3.3 Drinking water distribution system
Microorganisms can enter drinking water distribution systems as a result of inadequate treatment, passage through treatment barriers or through post-treatment contamination via intrusions, cross-connections or during construction or repairs. Biofilms and loose deposits in drinking water systems provide habitats that can support the survival, growth and dissemination of pathogenic microorganisms, particularly naturally-occurring pathogens (e.g., Legionella). Despite the detection of naturally-occurring bacterial pathogens in drinking water distribution systems, these systems are not expected to be a major source of exposure (LeChevallier, 2020). Maintaining an effective disinfectant residual is essential to manage risks. There is increasing recognition that a minimum disinfectant residual concentration greater than 0.2 mg/L is required to control microbiological (re)growth in the distribution system. Studies indicate that disinfectant residual concentrations in the order of 1.0 mg/L free chlorine (for systems that chlorinate) and 1.8 mg/L total chlorine (for systems that chloraminate) are required for controlling biofilm formation (Gagnon et al., 2008; Gillespie et al., 2014; Rand et al., 2014; LeChevallier et al., 2015a,b). Water utilities that regularly remove biofilms and loose deposits from their distribution systems may require lower concentrations. Cantor (2017) stressed the importance of removing accumulated material to meet water quality goals. Comprehensive, multi-parametric monitoring programs (e.g., disinfectant residual paired with temperature and biofilm formation rate) are recommended to confirm system-specific requirements (Health Canada, 2022).
The choice of secondary disinfectant for residual maintenance in the distribution system is a complex decision. Free chlorine and monochloramine possess different capabilities in terms of disinfectant power, reactivity with organic and inorganic material and biofilm penetration. Also, Legionella and mycobacteria have differing sensitivity to free chlorine and monochloramine. Thus, the choice of disinfectant residual should effectively balance concomitant water quality objectives related to: 1) microbial species (e.g., Legionella, mycobacteria); 2) water chemistry; 3) disinfection by-product concentrations; and 4) the complexity of the distribution system (Donohue et al., 2019a). Roth and Cornwell (2018) assessed the impact of increasing free chlorine residuals from 0.2 mg/L to 1.2 mg/L on disinfection by-product concentrations for 21 water utilities. The authors concluded that minimal impact was expected although system-specific assessments were recommended to avoid unintended consequences.
Additional information on managing microorganism survival and growth in drinking water distribution systems, and establishing multi-parametric monitoring programs, is found in these Health Canada publications: Guidance on Monitoring the Biological Stability of Drinking Water in Distribution Systems (Health Canada, 2022) and Guidance on Natural Organic Matter in Drinking Water(Health Canada, 2020a). Key distribution system operational and maintenance practices include:
- use proper construction materials;
- maintain an effective disinfectant residual and take preventive/corrective actions when low disinfectant residuals occur, particularly during warm water temperature conditions when biofilm growth accelerates;
- manage water age and the effects of temperature;
- minimize the potential for contaminant entry from external sources (e.g., maintain positive pressure, implement cross-connection/backflow control programs, practice strict hygiene during mains constructions and repairs);
- keep the distribution system clean by removing biofilm, loose deposits and sediment from watermains and storage facilities (e.g., use of appropriate flushing and cleaning techniques);
- conduct performance testing using multiple parameters (e.g., disinfectant residual, microbiological indicators, biological stability indicators, pH, pressure, temperature, turbidity); and
- provide operator training to assure the effectiveness of the water safety plan at all times.
A.3.4 Premise plumbing
Conditions in premise plumbing systems such as: 1) smaller pipe diameter; 2) increased temperature; 3) increased retention time; and 4) leaching of significant amounts of nutrients (e.g., carbon) from some pipe materials can lead to the growth of microorganisms, particularly opportunistic pathogens (e.g., Legionella) (Neu and Hammes, 2020). Thus, water utilities should educate their customers of the potential for water quality deterioration in premise plumbing.
Maintaining microbiological control in premise plumbing systems is a critical component of providing safe drinking water at the consumer’s tap. This is especially important in large buildings. Building owners/managers are responsible for managing water quality within their buildings and therefore should be aware of practices that reduce the risk of microorganism growth. Important elements of control strategies for plumbing systems include:
- limiting nutrient levels through an emphasis on system design and materials;
- minimizing areas of low flow/stagnation;
- keeping temperatures of cold and hot water systems outside of the ideal range for microorganism growth (e.g., cold water less than 20°C, hot water tank temperature greater than 60°C; hot water lines at distal points ideally greater than 55°C); and
- reducing the formation and transmission of contaminated aerosols from system components such as cooling towers, showers, faucets, hot tubs and humidifiers.
The increasing demand for conservation of energy, water and materials can have unplanned impacts on microbiological presence and growth. This is important for Legionella in particular but also has relevance for other opportunistic pathogens that can be problematic in premise plumbing. Changes to premise plumbing systems operations or features that involve the use of alternative water sources (e.g., reclaimed water, harvested rainwater), increased water age, reduced flows and changes to water temperatures in hot and cold building systems can have the unintended consequences of increasing the potential for growth of these pathogens (Bédard et al., 2016; Rhoads et al., 2016; NASEM, 2020).
Resources are available to assist building owners/managers develop a water management plan (WHO, 2007, 2011; HSE, 2013a, 2013b, 2014; PWGSC, 2016; CDC, 2017; ASHRAE, 2018). Other guidance documents provide standards and technical specifications for the design and installation of plumbing systems in buildings (NRCC, 2015a, 2015b; ASHRAE, 2018). In addition, building owners/managers should contact the responsible authority in the affected jurisdiction to confirm if specific requirements will apply to their system (e.g., cooling tower registry).
A.3.5 Roles and responsibilities
Water utilities and building owners/managers all have a role in effectively managing risks. Table 2 summarizes the key roles and responsibilities for stakeholders. Guidance is available to assist water utilities to develop education and outreach programs (Masters et al., 2019).
Water utility | Building owner/manager | |||
---|---|---|---|---|
Section | Source | Treatment | Distribution System | Building plumbing system |
Requirements | Source water protection plan | Appropriate treatment | Distribution system management plan | Building water management plan |
Objective | Protect the source to minimize risks to public health and reduce water treatment costs | Produce water that is of acceptable microbiological quality and is biologically stable to minimize water quality degradation in distribution and building/ premise plumbing systems | Establish policies and operational goals designed to protect water quality in the distribution system | Establish policies and operational goals designed to protect water quality in the building and premise plumbing system |
Education and outreach | Establish education and outreach programs to inform water consumers about the risks posed by water quality degradation from the source to the tap | n/a |
A.3.6 Impacts of a changing climate
Weather events such as heavy or intense rainfall, severe storms, flooding, dry spells/drought and hotter temperatures can contribute to increased human exposure to waterborne pathogens through various mechanisms (Levy et al., 2016; Nichols et al., 2018; Walker, 2018; Semenza, 2020; Calero Preciado et al., 2021) including:
- transport and loading of pathogens and nutrients to drinking water sources;
- overwhelming or damaging drinking water infrastructure;
- influencing pathogen survival and growth in source waters and drinking water distribution and plumbing systems;
- extending transmission seasons or geographical ranges for pathogens;
- diminishing availability of water resources;
- influencing human activities or behaviours (e.g., increases in water consumption and use of heating, ventilation and cooling systems).
These events are forecasted to increase in frequency and severity with climate change (Levy et al., 2016; Nichols et al., 2018; Walker, 2018). The Guidelines for Canadian Drinking Water Quality do not discuss all the potential climate scenarios that could impact water utilities and building water systems. The responsible authority should be consulted to discuss relevant forecast scenarios. Climate change impacts will heighten the importance of water quality monitoring, proper treatment process selection, day-to-day process control, distribution system operation, building water system management, and education and outreach programs. Thus, water utilities and building owners/managers should integrate the risks associated with climate change into their management strategies to maximize the reliability, robustness and resilience of their water systems. Guidance is available to assist water utilities (AWWA, 2021).
Part B. Technical information
B.1 Bacteria of gastrointestinal origin
B.1.1 Campylobacter spp.
B.1.1.1 Description
Campylobacter (Class: Epsilonproteobacteria) is a bacterial genus that contains over 30 recognized species, but only some have relevance for human health (Wagenaar et al., 2015; Backert et al., 2017; LPSN, 2019).Campylobacter jejuni (C. jejuni) and Campylobacter coli (C. coli) are the primary and secondary species of most relevance as causes of human gastrointestinal illness, accounting for greater than 90% of cases of human campylobacteriosis worldwide (Huang et al., 2015; Wagenaar et al., 2015). Other species are known to cause gastrointestinal illness, but their occurrence is rare or is associated with specific risk groups (e.g., immunocompromised individuals) or geographical areas (Wagenaar et al., 2015). Some Campylobacter species (spp.) have been associated with prenatal and neonatal infections and human periodontal disease (Backert et al., 2017; Huang et al., 2015).
Campylobacter spp. are Gram-negative, motile, curved or spiral rod-shaped cells (Percival and Williams, 2014b). They are fastidious and microaerophilic (require lower oxygen levels) bacteria that have a growth temperature of 30 to 45°C (optimum: 40-42°C) (Percival and Williams, 2014b; Wagenaar et al., 2015; Zautner and Masanta, 2016).
B.1.1.2 Health effects
Gastroenteritis caused by Campylobacter spp. includes a watery, profuse and sometimes bloody diarrhea occasionally accompanied by fever and abdominal pain (Backert et al., 2017; Percival and Williams, 2014b). Some severe infections may lead to hospitalization and can be life threatening, although fatalities are usually associated with the very young, very old, or patients with underlying disease or immune system deficiencies (Kvalsvig et al., 2014). Symptoms generally occur within one to five days of infection and the illness lasts less than seven to ten days (Backert et al., 2017). Shedding of the microorganisms in feces can continue for weeks following an episode of diarrhea; in most cases this ceases within four weeks (Percival and Williams, 2014b; Lee et al., 2013). Asymptomatic infections with Campylobacter spp. are also possible (Percival and Williams, 2014b). While Campylobacter spp. can cause illness in healthy individuals across all age groups, in developed countries, infections are more prevalent in young children, young adults and the elderly (Kaakoush et al., 2015; PHAC, 2018c). The doses of C. jejuni required to cause infection and illness are not fully understood. Human challenge studies have shown that for some strains, ingestion of several hundred bacteria can be sufficient to cause infection and illness (Black et al., 1988). Meta-analyses of C. jejuni pathogenicity data from challenge studies and foodborne outbreaks show that the doses required to cause infection and illness may be orders of magnitude lower (Teunis et al., 2005; 2018).
Post-infection complications associated with Campylobacter spp. illness include Guillain–Barré Syndrome and reactive arthritis, though these are relatively rare (Backert et al., 2017; Percival and Williams, 2014b). Campylobacter spp. infection may be associated with the development of inflammatory bowel diseases such as Crohn’s disease, ulcerative colitis and irritable bowel syndrome (Backert et al., 2017; Huang et al., 2015). A meta-analysis estimated that Campylobacter spp. cases develop long-term complications in the following proportions: Guillain–Barré Syndrome, 0.07% (95% confidence interval: 0.03-0.15%); reactive arthritis, 2.86% (95% CI: 1.40-5.61%); and irritable bowel syndrome, 4.01% (95% CI: 1.41-10.88%) (Keithlin et al., 2014b).Campylobacter spp. is the leading cause of bacterial gastrointestinal illness in Canada and other developed countries worldwide (Backert et al., 2017; Huang et al., 2015). Cases of campylobacteriosis in Canada and internationally are predominantly sporadic, with most illness associated with consumption of contaminated food (Huang et al., 2015; Wagenaar et al., 2015). In Canada, reported annual incidence rates (all causes) over the period from 2013-2017 ranged from 25.4 to 29.2 (median: 28.4) cases per 100,000 population (PHAC, 2019b). Infections (all sources) are more common in the summer months (Fleury et al., 2006; Lal et al., 2012; Kaakoush et al., 2015).
Illness caused by Campylobacter spp. is generally self-limiting, and antibiotics should only be prescribed in serious cases (Wagenaar et al., 2015). Campylobacter spp. are resistant to ciprofloxacin and azithromycin and have been classified as a “Serious Threat” by the Centers for Disease Control and Prevention (CDC, 2019a). The WHO and the Public Health Agency of Canada (PHAC) consider these microorganisms a medium to high priority for surveillance, research and public health attention (Garner et al., 2015; WHO, 2017b, PHAC, 2018a). No vaccines for Campylobacter spp. are available (Wagenaar et al., 2015).
B.1.1.3 Sources and exposure
Campylobacter spp. are zoonotic pathogens (i.e., transmitted from animals to humans) that are naturally found in the intestinal tract of a wide range of wild and domestic birds and mammals (Wagenaar et al., 2015; Backert et al., 2017). Poultry are considered the major reservoir (Wagenaar et al., 2015; Backert et al., 2017). Cattle, sheep and domestic pets are also important sources of the microorganisms (Wagenaar et al., 2015; Backert et al., 2017). Transmission of Campylobacter spp. occurs via the fecal-oral route, with the main pathways for exposure being contaminated food or water and direct contact with animals (Percival and Williams, 2014b; Wagenaar et al., 2015). Person-to-person transmission is uncommon (Percival and Williams, 2014b; Wagenaar et al., 2015). Important mechanisms for fecal contamination of drinking water sources (groundwater and surface water) are surface runoff containing livestock waste and sewage inputs (e.g., from wastewater discharges, leaking sanitary sewers) (Whiley et al., 2013). Intrusion of animal feces following heavy rainfall or snowmelt is a particularly important cause of contamination of vulnerable groundwater wells (Moreira and Bondelind, 2017).
Campylobacter spp. has been recognized as the enteric waterborne bacterial pathogen most frequently involved in drinking water outbreaks in developed countries (Moreira and Bondelind, 2017). According to United States (U.S.) data, Campylobacter spp. was identified as a causative or co-occurring agent in 11% of the drinking water outbreaks reported between 2001 and 2014 (the last year for which data is available). Outbreaks occurred in all months of the year, with the largest outbreaks occurring in the spring and summer months (CDC, 2004, 2006, 2008, 2011, 2013, 2015b, 2017d). Periods of higher risk for waterborne illness coincide with peak periods for precipitation-induced (e.g., rainfall, snowmelt) agricultural runoff (Sterk et al., 2013, Galanis et al., 2014).
The Walkerton outbreak of May 2000 is the most notable Canadian drinking water outbreak involving Campylobacter spp., with 105 confirmed cases and was also caused by pathogenic E. coli (163 confirmed cases) (O’Connor 2002a, 2002b). The severity of this outbreak (greater than 2300 estimated cases, 7 deaths) led to substantial reform of drinking water regulatory policy in Canada (O’Connor, 2002b; Holme, 2003). Notable large drinking water outbreaks involving Campylobacter spp. to have occurred worldwide since Walkerton include New Zealand (2016: greater than 1000 cases), Denmark (2010: 409 cases), Ohio, U.S. (2004: 1450 cases) and Finland (2001: 1000 cases) (Hrudey and Hrudey, 2004, Government Inquiry into Havelock North Drinking Water, 2017; Moreira and Bondelind, 2017). Drinking water outbreaks have largely been associated with small drinking water supplies (i.e., private wells or small community supplies) with contamination from infiltration of animal feces or wastewater and inadequate disinfection reported as the most frequent causes (Moreira and Bondelind, 2017). Private and small community water systems are recognized as being more likely to contribute to cases of human enteric illness than municipally-operated systems (Hrudey and Hrudey, 2004; Murphy et al., 2016; Butler et al., 2016). Using a Quantitative Microbial Risk Assessment (QMRA) approach, Murphy et al. (2016) estimated that roughly 5% of the total number of Canadian cases of Campylobacter spp. acquired annually might be attributable to consumption of water from contaminated small drinking water systems. In municipal drinking water systems, inadequate disinfection and post-treatment contamination via intrusions or cross-connections are the most common causes of Campylobacter spp. outbreaks (Moreira and Bondelind, 2017).
B.1.2 Escherichia coli/Shigella spp. (pathogenic strains)
B.1.2.1 Description
Escherichia coli (Class Gammaproteobacteria, Family: Enterobacteriaceae) are Gram-negative bacteria that are a member of the natural intestinal microbial community of humans and animals. They are facultatively anaerobic, motile or non-motile rod-shaped bacteria that can grow over a broad temperature range (7-45°C) with an optimal growth temperature of 37°C (Ishii and Sadowsky, 2008, Percival and Williams, 2014c). Whereas most strains (i.e., variants) of E. coli are harmless and function as indicators of fecal contamination, some have acquired virulence traits through gains and losses of genetic material (Croxen et al., 2013). These pathogenic E. coli strains can cause numerous human diseases including serious gastrointestinal infections, urinary tract and bloodstream infections and neonatal meningitis (Croxen et al., 2013; Percival and Williams, 2014c). Non-pathogenic E. coli strains and their role in drinking water risk management are addressed in Health Canada’s Guideline Technical Document on Escherichia coli (Health Canada, 2019e).
Pathogenic E. coli are broadly categorized into functional groups based on the mechanisms with which they interact with their target cells and cause symptoms. Different types can bind to, invade, or cause structural alterations of cells and produce specific types of toxins. There are six major groups of pathogenic E. coli that cause gastrointestinal infections: enterohaemorrhagic E. coli (EHEC), enterotoxigenic E. coli (ETEC), enteroinvasive E. coli (EIEC), enteropathogenic E. coli (EPEC), enteroaggregative E. coli (EAEC) and diffuse adherent E. coli (DAEC) (Croxen et al., 2013; Percival and Williams, 2014c). Categorization of pathogenic E. coli strains has previously been determined by biochemical testing and serotyping based on the classic Kauffmann-White classification scheme for surface O and H antigens (Croxen et al., 2013; Robins-Browne et al., 2016). Molecular methods have been developed that allow for more rapid detection and identification of the different pathogenic strains (Croxen et al., 2013; Robins-Browne et al., 2016). Serotyping information nevertheless remains helpful in outbreak investigations and disease surveillance (Robins-Browne et al., 2016). Additional pathogenicE. coli groups have been proposed, but these have not been completely defined. Comparative genomics studies have shown that these group designations are not always clear cut, and that there is considerable overlap in the virulence mechanisms possessed by different E. coli strains (Croxen et al., 2013).
Shigella spp. are closely related to E. coli but have historically been considered separate species. Shigella and EIEC strains are nearly indistinguishable based on their biochemical and serological properties (Croxen et al., 2013). Advanced molecular typing and sequencing analyses have demonstrated that Shigella spp. clearly belongs within the species E. coli, forming a single group with the EIEC (Croxen et al., 2013, Robins-Browne et al., 2016). A re-evaluation of the Shigella spp. classification may be required to take into account its genetic relationship to the Escherichia genus. The genus name Shigella spp. and disease name shigellosis (i.e., disease caused by Shigella spp.) are still used for historical purposes (Croxen et al., 2013). Conventionally, Shigella spp. has four major species ( S. dysenteriae, S. flexneri, S. boydii and S. sonnei ); with Shigella sonnei and Shigella flexneri being the most important in developed countries (Percival and Williams, 2014h).
Among the pathogenic E. coli, the EHEC (synonyms: shiga toxin-producing Escherichia coli and verotoxin-producing Escherichia coli (VTEC)) are of most concern to the drinking water industry (Percival and Williams, 2014c; Saxena et al., 2015). EHEC are the subset of E. coli that can produce one or more of the potent Shiga toxins and are considered to be highly pathogenic to humans. E. coli O157:H7 is the most prevalent EHEC serotype; however, other serotypes, i.e., O26, O45, O103, O111, O121, and O145, are also important causes of human illness (Croxen et al., 2013, Saxena et al., 2015; PHAC, 2018c).
B.1.2.2 Health effects
In developed countries, most E. coli illness occurs as sporadic cases or outbreaks associated with contaminated food and water or travel (Croxen et al., 2013; Saxena et al., 2015). In developing countries, enteric pathogenic E. coli are a significant cause of illness and mortality, particularly among children.
Enteric pathogenic E. coli/Shigella spp. cause diarrheal illness that can range in severity from mild and self-limiting to severe and life-threatening depending on the group and strain involved. The first symptom is watery diarrhea. This can be followed by bloody diarrhea in EHEC infections and occasionally during EIEC/Shigella spp. and EAEC infections (Croxen et al., 2013, Percival and Williams 2014c; 2014h). Additional symptoms can include nausea, vomiting, abdominal pain, fever, headache and muscle pain. Symptom onset generally occurs within one to three days of infection. The duration of diarrhea is usually one to two weeks, but can persist longer with some strains (Croxen et al., 2013; Percival and Williams, 2014c, 2014h). Infected individuals can become asymptomatic carriers capable of shedding the microorganisms in their feces for weeks to months after infection (Croxen et al., 2013; Percival and Williams, 2014c, 2014h). Doses required to cause infection are estimated to range from less than 100 to 1000 organisms for EHEC and EIEC/ Shigella spp. to greater than one million to ten billion organisms for the other groups (Kothary and Babu, 2001; Croxen et al., 2013, Percival and Williams, 2014c; 2014h).
EHEC illness is particularly concerning as it can progress to the serious and potentially life-threatening hemolytic uremic syndrome (HUS), which results in decreased blood cell and platelet counts and acute kidney failure. A meta-analysis showed that HUS was the predominant long-term complication following cases of E. coli O157 illness, with an estimated rate of occurrence between 4-17% (Keithlin et al., 2014a). HUS can also lead to further long-term effects in the pancreas, gastrointestinal system and central nervous system (Spinale et al., 2013). Complications arising from non-EHEC infections are uncommon (Croxen et al., 2013). A link between infections with some pathogenic E. coli types (i.e., DAEC and some invasive E. coli) and chronic intestinal disorders such as irritable bowel syndrome and Crohn’s disease has been suggested (Croxen et al., 2013). In developed countries, enteropathogenic E. coli can cause gastrointestinal infections in healthy individuals in all age groups. Young children and the elderly are at higher risk of experiencing illness and complications as a result of infection (Percival and Williams, 2014c, 2014h; Gargano et al., 2017).
EHEC and Shigella spp. are among the leading causes of bacterial gastrointestinal illness in Canada, the U.S. and Europe (Scallan et al., 2011; CDC, 2018; ECDC, 2018a; PHAC, 2019b). Case reports and outbreaks of E. coli-related diarrheal illness and shigellosis in North America have mostly been tied to food, and travel-related exposures, though waterborne exposure remains an important cause of infections (Croxen et al., 2013; PHAC, 2018c). Reported annual incidence rates in Canada for verocytotoxigenic E. coli infection and shigellosis (all causes) over the period from 2013 to 2017 were 1.78-2.24 (median: 1.82) cases per 100,000 persons and 1.94-2.53 (median: 2.28) cases per 100,000 persons, respectively (PHAC, 2019b). Seasonal trends in EHEC and Shigella spp. infections (all sources) have generally been observed worldwide, with more cases occurring in summer to early fall (Fleury et al., 2006; PHAC, 2010; Lal, 2012).
In most cases, E. coli diarrheal infections are self-limiting. Treatment generally involves oral rehydration to maintain fluid and electrolyte balance. Antibiotics can be prescribed in severe cases with some strains of E. coli, but are not normally recommended for EHEC infections, as they can stimulate Shiga toxin production, increasing the risk of HUS (Croxen et al., 2013).
The CDC, WHO and PHAC have identified carbapenem-resistantE. coli and extended spectrum β-lactamase (ESBL)-producing E. coli as public health threats of serious to critical importance (CDC 2019a; WHO, 2017b, PHAC, 2018a). ESBL-producing E. coli are generally resistant to many antibacterial drugs; for persons with severe infections with these strains, carbapenems are one of the main treatment options. Resistance to carbapenems means resistance to one of the last available treatment options (CDC 2019a, WHO, 2017b). Pathogenic E. coli strains resistant to ESBL antibiotics and carbapenems have been recovered from humans and animals (Mir and Kudva, 2018). Shigella spp. resistant to ciprofloxacin and azithromycin also have been designated as a “Serious Threat” level by the CDC, and have been designated as a low to medium priority for research and surveillance by PHAC and the WHO (CDC, 2019a, Garner et al., 2015; WHO, 2017b). As Shigella spp. resistance to first-line drugs has increased, treatment has shifted to reliance on these two drugs for treating resistant infections (CDC, 2019a; WHO, 2017b). A vaccine based on the cholera toxin (which is structurally similar to the ETEC heat-labile toxin) has been licensed for use to protect against ETEC-associated traveller’s diarrhea (Croxen et al., 2013; O’Ryan et al. 2015). More data is required to determine the effectiveness of this and other candidate vaccines for ETEC (O’Ryan et al. 2015). No vaccines are available for other E. coli groups (Croxen et al., 2013).
B.1.2.3 Sources and exposure
Humans are the primary reservoir for the EPEC, ETEC and EAEC groups and the only known reservoir for EIEC/Shigella spp. (Croxen et al., 2013). EHEC are important zoonotic pathogens. Ruminants, particularly cattle, are the primary reservoir for EHEC; humans are a secondary reservoir (Croxen et al., 2013, Percival and Williams, 2014c). Animals (e.g., cattle, dogs, sheep, rabbits) are also a reservoir for certain EPEC strains (Croxen et al., 2013). Transmission of pathogenic E. coli occurs through the fecal-oral route and the main routes of infection are contaminated food or water, person-person spread and direct contact with animals. Surface runoff and sewage inputs are important mechanisms for fecal contamination of drinking water sources—much the same as those discussed for Campylobacter spp. (see Section B.1.1) (Hrudey and Hrudey, 2004; Moreira and Bondelind, 2017).
In the U.S., pathogenic E. coli (largely E. coli O157:H7) was identified as a causative or co-occurring agent in roughly 4% of the drinking water outbreaks reported over the period between 2001 and 2014 (the last year for which data is available) (CDC, 2004, 2006, 2008, 2011, 2013, 2015b, 2017d). Most E. coli drinking water outbreaks have been associated with small drinking water supplies (i.e., private wells or small community supplies) (Craun et al., 2010; CDC, 2011, 2013, 2015b, 2017d). QMRA estimates suggest that consumption of untreated or inadequately treated water from small drinking water supplies may be responsible for 4% of all cases of E. coli O157 illness in Canada (Murphy et al., 2016). The Walkerton outbreak of May 2000 is the most notable Canadian drinking water outbreak involving pathogenic E. coli [and Campylobacter (see Section B.1.1.3)], resulting in an estimated 2300 total cases, 163 confirmed cases of E. coli O157, 27 HUS cases and seven deaths (O’Connor 2002a, 2002b). Since the Walkerton outbreak, large drinking water outbreaks involving pathogenic E. coli have occurred in Missouri, U.S. (2010: 28 cases, 0 deaths) and Korea (2015: 188 cases, 0 deaths), (Hrudey and Hrudey, 2004; Missouri Department of Health and Senior Services, 2011; Park et al., 2018). Shigella spp. is rarely linked to drinking water outbreaks (Hrudey and Hrudey, 2004; Craun et al., 2010). Three reports of drinking water outbreaks involving Shigella spp. have been recorded in the U.S. between 2001 and 2014, all associated with irregular sources of drinking water (pond, lake water, commercially bottled water system) (CDC, 2006, 2011, 2015b).
Investigations of drinking water-related illness in Canada have found that the majority of waterborne disease outbreaks occur in the spring and summer (Schuster et al. 2005, Thomas et al., 2006; Wilson et al., 2009). Weather variables (e.g., snowmelt, warmer temperatures, alternation between dry conditions and intense rain events) and increased human and animal activities in the watershed (e.g., grazing animals and land application of fecal wastes in agriculture) have been proposed as factors contributing to these trends (Tyrell and Quinton, 2003; Thomas et al., 2006; Wilson et al., 2009; Quilliam et al., 2011). Heavy rainfall causing flooding contributed to the Walkerton, Ontario E. coli O157:H7 and Campylobacter spp. outbreak of 2000 (O’Connor, 2002a).
B.1.3 Helicobacter pylori
B.1.3.1 Description
Helicobacter pylori (H. pylori, Class: Epsilonproteobacteria) is a pathogenic bacterium that can colonize the human stomach and is responsible for causing gastrointestinal diseases which can include gastritis, peptic ulcers and gastric cancer (Percival and Williams, 2014d; Posteraro et al., 2015). Helicobacter are closely related to the genus Campylobacter (Percival and Williams, 2014d). Over 20 different Helicobacter species have been determined by gene sequencing (Percival and Williams, 2014d; Posteraro et al., 2015). H. pylori is the predominant pathogenic species of the genus, accounting for the vast majority of human infections. Some other Helicobacter species have occasionally been associated with human gastrointestinal illness (Percival and Williams, 2014d).
H. pylori are Gram-negative, motile, fastidious and microaerophilic (require lower oxygen levels) bacteria that grow over the temperature range of 30-42°C (optimum: 37°C) (Mégraud and Lehours, 2007; Posteraro et al., 2015). They are not acidophilic (acid-loving) bacteria, but possess mechanisms that enable the bacteria to tolerate the acid conditions of the human stomach. H. pylori have two morphological forms: a spiral (S-shaped) rod form and a viable but non-culturable (VBNC) coccoid form that is adopted under conditions of environmental stress. The VBNC form is an important component of the organism’s survival strategy; however, its role in pathogenesis is unknown (Percival and Williams, 2014d).
B.1.3.2 Health effects
The vast majority of H. pylori infections are asymptomatic (Percival and Williams, 2014d). H. pylori infection can cause a chronic and superficial gastritis, and some infections develop into peptic (i.e., duodenal or gastric) ulcers (Posteraro et al., 2015). Symptoms of gastritis and ulcers include nausea, abdominal pain, heartburn and bleeding (Percival and Williams, 2014d; Posteraro et al., 2015). In a very small fraction of the infected population, infections can develop into gastric cancer. H. pylori has been classified by the International Agency for Research on Cancer (IARC) as carcinogenic to humans (IARC, 2014), and the organism is considered to be the single most common cause of gastric cancer worldwide (Percival and Williams, 2014d; Posteraro et al., 2015). The infectious dose of H. pylori is not known. Challenge studies suggest it is less than 10,000 cells (Solnick et al., 2001; Graham et al., 2004); however, evidence from case reports of illness suggests the dose could be orders of magnitude lower (Langenberg et al., 1990; Matysiak-Budnik et al., 1995).
The varying health outcomes of H. pylori infection seem to be related to human genetic variability, environment and dietary factors, and differences in strain virulence (Brown, 2000; Posteraro et al., 2015). Since the majority of persons do not develop clinical disease, it can be difficult to determine when infection occurs (Brown, 2000). People living in low socioeconomic status, poor hygiene or sanitary conditions and crowded or high-density living conditions are associated with higher prevalence of H. pylori infections (Brown, 2000). Rates of infection are higher in developing countries and in at-risk populations, with most infections being acquired during childhood in these locations (Brown, 2000, Posteraro et al., 2015). Childhood rates in developed countries are lower and may be decreasing with improvements to sanitary practices (Brown, 2000). H. pylori have been cited as the most prevalent bacterial pathogen of humans (Posteraro at al., 2015). Roughly one half of the world’s population is infected with H. pylori (Percival and Williams, 2014d). Rates of asymptomatic H. pylori infections vary widely by geographical area but are broadly estimated to fall in the range from 20 to 50% in developed regions and from 50 to >70% in developing countries (Brown, 2000; Hooi et al., 2017; Zamani et al. 2018). The rates of H. pylori infections in Canada are not well understood as they are not a reportable illness. Studies of H. pylori infections in Ontario among adults 50 to 80 years of age and Canadian children with upper gastrointestinal symptoms have reported rates of 23.1% and 7.1%, respectively (Naja et al., 2007; Segal et al., 2008). Higher rates (>40%) have been reported among First Nations populations in Canada (Bernstein et al., 1999; Sethi et al., 2013; Fagan-Garcia et al., 2019).
Once colonized by H. pylori, infections can be lifelong unless intensive antimicrobial therapy is provided (Percival and Williams, 2014d). Eradication of H. pylori has been shown to be the definitive cure for duodenal and most gastric ulcers (Percival and Williams, 2014d). Helicobacter resistant to clarithromycin and multi-drug-resistant Helicobacter have been identified as a medium to high priority for research and the development of new antibiotic strategies by PHAC and the WHO (Garner et el., 2015; WHO, 2017b). No effective vaccines against H. pylori infection have been developed (Posteraro et al., 2015).
B.1.3.3 Sources and exposure
Hosts for H. pylori include humans, domestic cats and non-human primates (e.g., old world macaques) (Percival and Williams, 2014d). The human stomach is considered the major reservoir (Percival and Williams, 2014d). Domestic cats are also thought to be a source of the organism relevant for human infections (Percival and Williams, 2014d).
The principal means through which H. pylori infections are acquired is unclear. Person-to-person transfer is presumed to be the most likely route of transmission via fecal-oral, gastric-oral or oral-oral routes (Percival and Williams, 2014d; Posteraro et al., 2015). Direct contact with domestic cats is also thought to be a pathway for infection; however, there is no confirmed data on transmission from animals to humans (Brown, 2000). Consumption of contaminated drinking water is suspected as a potential source of infection. Infection occurring through multiple transmission pathways is likely (Percival and Williams, 2014d). Attempts to culture H. pylori from environmental water samples have mostly been unsuccessful, and this absence of cultured data has limited epidemiological and risk assessments (Percival and Williams, 2014d). Evidence for a waterborne transmission comes largely from epidemiological studies conducted in developing countries (Percival and Williams, 2014d). Further support for water as vehicle of transmission has been provided by culture of H. pylori in feces of infected individuals; detection of H. pylori by molecular methods in drinking water supplies; and the finding of an association between H. pylori in untreated groundwater supplies and clinical infection in individuals drinking the water (Baker and Hagerty, 2001). In countries with adequate drinking water treatment, drinking water is unlikely to be a significant source of infection (Percival and Williams, 2014d). Nevertheless, further research on the role of water in the spread of H. pylori infections is needed. Studies on the detection of H. pylori in municipal drinking water supplies have been limited. Surveys of H. pylori presence in water and biofilm samples collected from the plumbing of private residences and public facilities receiving municipal water have reported detection of the microorganism by polymerase chain reaction (PCR) detection in 4-64% of the locations sampled (Watson et al., 2004; Santiago et al., 2015; Richards et al., 2018). H. pylori are not a recognized cause of waterborne outbreaks (Percival and Williams, 2014d).
B.1.4 Salmonella spp.
B.1.4.1 Description
Salmonella (Class Gammaproteobacteria, Family: Enterobacteriaceae) is a large and diverse group of bacteria that can cause gastrointestinal infections in animals and humans. Molecular methods have shown that the genus consists of only two species,Salmonella enterica and Salmonella bongori(Percival and Williams, 2014g; Graziani et al., 2017). Salmonella enterica is further divided into six subspecies and contains the majority of the over 2500 serotypes that have been identified (Grimont and Weill, 2007; Percival and Williams, 2014g). Early in Salmonella identification, serotypes were treated as species and given names that reflected the host or disease it was associated with or, later, the geographic location where it was found (Grimont and Weill, 2007). When the current taxonomic structure of Salmonella was established, these names had become so familiar that they have been maintained, substituting for the O and H group naming structure that is more commonly used with other bacterial species (Grimont and Weill, 2007).
Salmonella of human importance are broadly categorized into two main groups according to the type of disease they cause. The typhoidal Salmonella (S. enterica serotype Typhi and S. enterica serotype Paratyphi) are the causative agents of enteric fever (also known as typhoid or paratyphoid fever), a serious and life-threatening illness (Sanchez-Vargas et al., 2011). The non-typhoidal Salmonella are a large group containing the remainder of the S. enterica serotypes which cause gastrointestinal illness of varying severity (Sanchez-Vargas et al., 2011). In developed countries non-typhoidal Salmonella are the most important as food and waterborne pathogens (Sanchez-Vargas et al., 2011; Percival and Williams, 2014g). Salmonella serotype Enteritidis (S. Enteritidis) and Salmonella serotype Typhimurium (S. Typhimurium) are the serotypes most commonly encountered as causes of human infections (Sanchez-Vargas et al., 2011).
Salmonella are Gram-negative, facultative anaerobic, predominantly motile rod-shaped bacteria that can grow over the temperature range of 5-47°C, and optimally at 35-37°C (Graziani et al., 2017).
B.1.4.2 Health effects
Salmonella infections follow different courses of disease depending on whether the serotype is typhoidal or non-typhoidal (Sanchez-Vargas et al., 2011). Non-typhoidal Salmonella cause a gastroenteritis characterized by diarrhea, fever and abdominal pain (Percival and Williams, 2014g, Graziani et al., 2017). Symptoms occur within 12-72 hours of infection and the illness may last four to seven days. In severe cases, infection can spread to other parts of the body (e.g., blood, urine, joints, brain) and can be life-threatening (Percival and Williams, 2014g; Sanchez-Vargas et al., 2011). Children have the highest incidence of Salmonella infections (Christenson, 2013; PHAC, 2018c). Severe infections and fatal cases are rare and are more commonly reported among the very young, the very old and those with compromised immune systems or underlying illness (Sanchez-Vargas et al., 2011; Dekker and Frank, 2015). A meta-analysis of non-typhoidal Salmonella cases developing long-term complications estimated the proportion developing reactive arthritis at 5.8% (95% CI: 3.2-10.3%) and irritable bowel syndrome at 3.3 % (95% CI: 1.6-6.6%) (Keithlin et al., 2015). Estimates for other outcomes (e.g., HUS, Guillain–Barré Syndrome) were impeded by a lack of data (Keithlin et al., 2015). Typhoidal Salmonella cause enteric fever, an invasive and systemic disease which involves high fever, vomiting, headaches and numerous potentially fatal complications (Sanchez-Vargas et al., 2011). Enteric fever predominantly occurs in developing countries. In developed countries the incidence of enteric fever is infrequent and mostly related to travel (Sanchez-Vargas et al., 2011). The dose required to cause infection varies depending on the serotype involved and the susceptibility of the host. Data suggests that the infective dose (non-typhoidal Salmonella) can range from a low of less than 100 organisms to a high of 100,000 to 10 billion organisms (Kothary and Babu, 2001).
Salmonella is the second-leading cause of bacterial gastrointestinal illness in Canada, the U.S. and Europe (Scallan et al., 2011; CDC, 2018; ECDC, 2019; PHAC, 2019b). In Canada, reported annual incidence rates of salmonellosis (all sources) in 2013-2017 ranged from 17.6 to 21.7 (median: 21.38) cases per 100,000 population (PHAC, 2019b). Cases of illness are predominantly sporadic, with most illness associated with consumption of contaminated food. Peak incidence of disease (all sources) occurs in the summer and fall (Fleury et al., 2006; Lal et al., 2012).
Infections with non-typhoidal Salmonella are generally self-limiting, and treatment involves fluid and electrolyte replacement (Percival and Williams, 2014g). Antibiotics can be prescribed in severe cases where there is increased risk of infection spread (Sanchez-Vargas et al., 2011; Percival and Williams, 2014g). No human vaccines are currently available for non-typhoidal Salmonella infections (Sanchez-Vargas et al., 2011). The CDC, WHO and PHAC have categorized non-typhoidal Salmonella resistant to ciprofloxacin, ceftriaxone or multiple classes (e.g., >3) of drugs as priority and “High-level Threats” (CDC 2019a; WHO, 2017b, PHAC, 2018a). In developed countries, antibiotic resistance in Salmonella has generally followed trends in the use of antimicrobials in food-producing animals (McDermott et al., 2018). The most frequent types of resistances observed are for older antimicrobials (e.g., tetracycline, sulfonamides, streptomycin) (McDermott et al., 2018). Declines in resistance rates for critical drugs for animals and humans (third generation beta-lactam antibiotics, ciprofloxacin) have been reported in the U.S. and Canada and are consistent with policies limiting their use in agriculture (McDermott et al., 2018; PHAC, 2018a).
B.1.4.3 Sources and exposure
Non-typhoidal Salmonella are zoonotic pathogens. Chicken, pigs, turkey and cattle are considered the most important reservoirs (Graziani et al., 2017). Other animals (dogs, birds, rodents, reptiles) and humans (infected individuals and asymptomatic carriers) are also recognized as sources (Percival and Williams, 2014g; Graziani et al., 2017). Humans are the only known reservoir for the typhoidal Salmonella serotypes (Percival and Williams, 2014g).
Salmonella are spread by fecal-oral transmission. For the non-typhoidal serotypes, contaminated food is the most common pathway for infection; and person-to-person contact and direct contact with animals are significant exposure pathways (Percival and Williams, 2014g; Graziani et al., 2017). Ingestion of contaminated water is a recognized route for infection of non-typhoidal Salmonella(Graziani et al., 2017). For sources of contamination important to drinking water, seeCampylobacter spp. (see Section B.1.1). Non-typhoidal Salmonella are very rarely linked to drinking water outbreaks (CDC, 2004, 2006, 2008, 2011, 2013, 2015b, 2017d; Hrudey and Hrudey, 2004).
B.1.5 Yersinia spp.
B.1.5.1 Description
The genus Yersinia (Class: Gammaproteobacteria, Family: Enterobacteriaceae) contains approximately 20 bacterial species, with only three recognized as human pathogens. Two species (Yersinia enterocolitica, Yersinia paratuberculosis) are recognized as food or waterborne enteropathogens that can cause acute gastroenteritis of mild to high severity (Percival and Williams, 2014i; Fredriksson-Ahomaa, 2015). Yersinia enterocolitica can be divided into six biotypes differentiated by physiochemical and biochemical tests, and into more than 30 serotypes based on variations in their surface O antigens (Sabina et al., 2011; Fredriksson-Ahomaa, 2015). Human infections have traditionally been associated with certain biotype and serotype combinations. Types 1b:O8, 2:O5,27, 2:O9, 3:O3, 4:O3 are most commonly associated with human disease worldwide (Todd, 2014; Fredriksson-Ahomaa, 2015, 2017). Y. paratuberculosis is more closely related to the plague bacteria (Yersinia pestis) than Y. enterocolitica, and is a less common cause of human infections (Todd, 2014). For Y. paratuberculosis there are over 20 serotypes based on O antigen variations, all of which are pathogenic (Percival and Williams, 2014i).
Members of the genus Yersinia are Gram-negative, motile, facultatively anaerobic, rod to coccobacilli-shaped cells that are able to grow at temperatures over the range of 4-43°C (optimum: 28-30°C) (Todd, 2014; Fredriksson-Ahomaa, 2015).
B.1.5.2 Health effects
Enteropathogenic Yersinia are enteroinvasive microorganisms which colonize and invade colon epithelial cells, causing diarrhea and inflammatory reactions (Percival and Williams, 2014i; Todd, 2014). Disease caused by Y. enterocolitica or Y. paratuberculosis is commonly referred to as yersiniosis (Fredriksson-Ahomaa, 2015). Symptoms of yersiniosis can differ depending on the age and immunity of the person infected, the strain involved and the infective dose (Todd, 2014). Diarrhea (often bloody), fever and abdominal pain are the most common symptoms in infants and young children under 5 years of age (Fredriksson-Ahomaa, 2015). In older children and adults, fever and right-sided abdominal pain may be the predominant symptoms and can be confused with appendicitis (Todd, 2014; Fredriksson-Ahomaa, 2015). Symptoms occur 1 to 11 days after exposure and can persist 1 to 3 days or longer (Todd, 2014; Fredriksson-Ahomaa, 2015). Asymptomatic infections with Y. enterocolitica and Y. paratuberculosis have been reported; and the pathogens can continue to be shed in feces for weeks after symptoms have ceased (Todd, 2014). Occasionally in severe cases, the bacteria can enter the lymph nodes and infection can be further spread by the bloodstream (Percival and Williams, 2014i; Fredriksson-Ahomaa, 2015). Complications from infections are uncommon, but can include joint pain (reactive arthritis) and skin rash (Percival and Williams, 2014i; Fredriksson-Ahomaa, 2015). Other symptoms less frequently associated with enteropathogenic Yersinia infection are various inflammatory reactions resulting from infection spread to other parts of the body (e.g., liver, spleen, lung, heart, brain, bones) (Percival and Williams, 2014i, Todd, 2014). Young children are more likely to become ill from infection with enteropathogenic Yersinia (Todd, 2014; PHAC, 2018c). Severe infections and fatal cases are rare and are typically observed in the elderly and immunosuppressed individuals (Todd, 2014). The infective dose is estimated to range between 10 thousand and 1 billion organisms for both Y. enterocolitica and Y. paratuberculosis (Todd, 2014); however, the dose is likely lower for immunosuppressed individuals (Fredriksson-Ahomaa, 2017).
Enteropathogenic Yersinia are a significant cause of bacterial gastrointestinal illness in Canada, the U.S. and Europe (PHAC 2019a; CDC 2018; ECDC, 2018b). National incidence data is not available as yersiniosis is a notifiable disease only in the province of Alberta (PHAC, 2019a). However, Canadian sentinel surveillance data from three locations (BC, AB and ON), reported endemic incidence rates of 10.16, 1.77 and 0.00 per 100,000 population respectively in 2018 (PHAC, 2019a). The majority of cases of Yersinia-associated illness are caused by Y. enterocolitica and are linked to the consumption of contaminated food (Todd, 2014; Fredriksson-Ahomaa, 2015; PHAC, 2018c). Generally, Yersinia infections are more frequently observed during the winter months (Todd, 2014; Fredriksson-Ahomaa, 2015).
Infections with Y. enterocolitica or Y. paratuberculosis are normally self-limiting, with treatment provided only in severe cases involving systemic infection or bacteremia (Todd, 2014; Fredriksson-Ahomaa, 2015). No human vaccines are available.
B.1.5.3 Sources and exposure
Pathogenic and non-pathogenic Yersinia spp. can be found in the gut of a variety of wild and domestic animals (Percival and Williams, 2014i; Fredriksson-Ahomaa, 2015). Pigs are the major reservoir of pathogenic strains of Y. enterocolitica; and ruminants (e.g., cattle, sheep, goats), dogs and cats are also important sources of the pathogen (Todd, 2014; Fredriksson-Ahomaa, 2015). Rodents and birds are considered the major reservoirs for Y. paratuberculosis (Todd, 2014; Fredriksson-Ahomaa, 2015). Pathogenic Yersinia spp. are zoonotic, and thus can be transmitted from animals to humans via the fecal-oral route (Fredriksson-Ahomaa, 2015). Contaminated food sources are the most significant pathway for infections (Todd, 2014; Fredriksson-Ahomaa, 2015). Consumption of contaminated water and direct contact with animals are also important infection pathways (Todd, 2014; Fredriksson-Ahomaa, 2015). Person-to-person transmission is possible, but rare (Todd, 2014; Fredriksson-Ahomaa, 2015).
In most studies, it is the non-pathogenic species or strains that are more frequently isolated (Brennhovd et al., 1992; Cheyne et al., 2009; Schaffter and Parriaux, 2002). The low isolation frequency in environmental samples may be due to limitations in the sensitivity of culture-based methods (Fredriksson-Ahomaa and Korkeala, 2003). Cheyne et al. (2010) found that using PCR methods, Yersinia virulence genes were detected in 21-38% of samples taken from a heavily impacted river watershed that was used as a source for a drinking water treatment system.
Yersinia spp. have very rarely been linked to drinking water outbreaks. According to U.S. data for 2001-2014 (the last year for which data is available), Yersinia enterocolitica was associated with one drinking water outbreak as a co-occurring agent with Campylobacter jejuni (CDC, 2004, 2006, 2008, 2011, 2013 2015b, 2017d). A contaminated untreated non-community groundwater supply was identified as the cause of the outbreak (CDC, 2004).
B.1.6 Analytical methods
Standard methods are available for the detection of Campylobacter spp., pathogenic E. coli/Shigella spp., Salmonella spp. and Yersinia spp. in drinking water (APHA et al., 2017; ISO, 2019). The procedures for isolating and identifying these bacteria commonly involve steps such as enrichment and/or separation, plating, colony screening and identification using biochemical tests, serological techniques, molecular methods or commercial kits (e.g., for toxins) (APHA et al., 2017; ISO 2019).
No standard methods for the detection of viable Helicobacter spp. in water have been established (Percival and Williams, 2014d, APHA et al., 2017). Methods for the detection of H. pylori in water environments involve the use of culture-independent molecular techniques such as PCR or fluorescent in-situ hybridization (Watson et al., 2004; Percival and Williams, 2014d; Santiago et al., 2015; Richards et al., 2018).
For a review of methods used in research settings, please refer to: Ramírez-Castillo et al., 2015; Kennedy and Wilkinson, 2017; Gorski et al., 2019; Li et al., 2020.
B.1.7 Treatment considerations
When properly designed and operated, physical removal technologies—chemically-assisted, slow sand, diatomaceous earth and membrane filtration or an alternative proven technology—and primary disinfection methods—chlorine chlorine dioxide, ozone and ultraviolet (UV) light—commonly used in drinking water treatment are very effective in reducing or inactivating the enteric bacteria described in the preceding sections (LeChevallier and Au., 2004). Monochloramine should not be used for primary disinfection due to its low oxidation potential; monochloramine is recommended only for secondary disinfection (i.e., to maintain a disinfectant residual in the distribution system) (Health Canada, 2019b).
The CT requirements for chemical disinfectant inactivation of these bacteria are comparable to those for E. coli and less than those required for enteric protozoa and enteric viruses (Sobsey, 1989; Lund, 1996; Johnson et al., 1997; Rice et al., 1999; Baker et al., 2002; LeChevallier and Au, 2004; Rose et al., 2007; Wojcicka et al., 2007; Chauret et al., 2008; Rasheed et al., 2016; Jamil et al., 2017; Health Canada, 2019b, 2019c, 2020c). The UV dose (intensity × time) requirements for inactivation of these microorganisms are similarly comparable to those for E. coli and enteric protozoa, and less than those needed for many enteric viruses (Sommer et al., 2000; Zimmer and Slawson, 2002; Smeets et al., 2006; Hayes et al., 2006; Zimmer-Thomas et al., 2007; Hijnen et al., 2011; Health Canada, 2019b, 2019c, 2020c).
General operational and maintenance practises for the control of microbial growth and survival in drinking water distribution and plumbing systems are outlined in Part A (LeChevallier and Au, 2004; Friedman et al., 2017). These are necessary to manage biofilms which can provide a habitat for the survival of fecal pathogens that may have passed through drinking water treatment barriers or entered the distribution system directly via an integrity breach (Leclerc, 2003).
For residential-scale systems and private wells, regular physical inspection to identify deficiencies and testing of the water system (e.g., for E. coli and total coliforms) to confirm the microbiological quality of the water are important. General guidance on well construction, maintenance, protection and testing is typically available from provincial/territorial jurisdictions. Well owners can also consult the Be Well Aware series for information (Health Canada, 2019a). Where treatment is necessary, Health Canada recommends that consumers use devices certified by an accredited certification body as meeting the appropriate NSF International (NSF)/American National Standards Institute (ANSI) drinking water treatment unit standards (NSF/ANSI, 2018, 2019, 2020). Certification organizations provide assurance that a product conforms to applicable standards and must be accredited by the Standards Council of Canada (SCC). An up-to-date list of accredited certification organizations can be obtained from the SCC (2020).
B.1.8 International considerations
No drinking water guidelines for the enteric bacterial pathogens Campylobacter spp., enteric pathogenic E. coli/Shigella spp., Helicobacter pylori, Salmonella spp. and Yersinia spp. have been established by the WHO, the EU, the US EPA or the Australian National Health and Medical Research Council (NHMRC, NRMMC 2011; WHO, 2017a; European Commission, 2020; US EPA, 2021a). Similar to Health Canada’s guidance document, the WHO and Australian drinking water guidelines contain fact sheets that provide information on waterborne pathogens of concern.
B.2 Naturally-occurring pathogens
B.2.1 Bacteria
B.2.1.1 Aeromonas spp.
B.2.1.1.1 Description
The bacterial genus Aeromonas (Class: Gammaproteobacteria) has a complex taxonomy. Around 30 species have been associated with the genus and potential new species continue to be described, although not all have been universally accepted (Janda and Abbott, 2010; Percival and Williams, 2014a; LPSN, 2019). The difficulties with Aeromonas identification arises from the lack of clear-cut phenotypic characteristics and the absence of a consistent typing scheme for distinguishing species. As a result, the use of biochemical and molecular methods is required for an accurate classification. Clinically relevant Aeromonas spp. are opportunistic pathogens that have been linked to a variety of intestinal and extra-intestinal diseases and syndromes (Janda and Abbott, 2010, Liu, 2015). Fourteen species have been implicated in human illness, however most human infections (85%) are caused by strains of four species: A. hydrophila, A. caviae, A. veronii (biotype sobria) and A. trota (Percival and Williams, 2014a; Liu, 2015; Bhowmick and Battacharjee, 2018).
Aeromonads are Gram-negative, facultatively anaerobic, non-spore-forming rod-shaped bacteria (Janda and Abbott, 2010; Percival and Williams, 2014a). Strains associated with human infections grow optimally at temperatures of 35-37°C, although many strains can grow in 4-42°C (Janda and Abbott, 2010; Percival and Williams, 2014a; Liu, 2015).
B.2.1.1.2 Health effects
Gastroenteritis is the most commonly encountered disease associated with Aeromonas infection (Janda and Abbott, 2010). Forms of the disease range from a watery enteritis accompanied by low-grade fever, vomiting and abdominal pain (most common) to a dysenteric form involving bloody stools (rare), to a cholera-like illness (very rare) (Janda and Abbott, 2010, Liu, 2015). Aeromonas spp. are an infrequent cause of traveller’s diarrhea and they can also be associated with a subacute or chronic intestinal infection (Janda and Abbott, 2010, Liu, 2015).
The time between infection and symptom onset is one to two days for Aeromonas-associated traveller’s diarrhea (Janda and Abbott, 2010). Subacute cases of diarrhea are defined as those lasting from two weeks to two months, whereas chronic cases persist for longer periods (Janda and Abbot, 2010). Complications that have been associated with more severe cases of Aeromonas gastroenteritis include ulcerative colitis, haemolytic uremic syndrome and inflammatory bowel disease (Janda and Abbott, 2010, Liu, 2015). The dose of Aeromonas spp. necessary to cause gastrointestinal infection is not clear. The only published challenge study showed that only 2/5 strains produced infection (14/57 individuals) and diarrhea (2/57 individuals) at high concentrations (ten thousand to ten billion colony forming units (CFU)) (Morgan et al., 1985). Data provided from foodborne outbreaks that have been observed suggests that the concentration required to cause infection could be orders of magnitude lower for some Aeromonas strains (Teunis and Figueras, 2016).
Skin and soft tissue infections are the second most common forms of Aeromonas-related disease. Aeromonas spp. can be associated with a variety of infections ranging from mild irritations (e.g., pus-filled lesions) to serious or life-threatening infections such as cellulitis or flesh-eating disease (Janda and Abbott, 2010; Bhowmick and Battacharjee, 2018). Aeromonads have also been implicated in blood-borne infections, which largely arise through the transfer of bacteria from gastrointestinal tract or wound infections. Common features associated with these infections are fever, jaundice, abdominal pain and septic shock (Janda and Abbott, 2010). Other less frequent diseases linked to Aeromonas infection include respiratory tract, urogenital tract and ocular infections (Janda and Abbott, 2010). High mortality rates have been observed with Aeromonas septicemia and severe wound infections in high-at-risk individuals (Janda and Abbot, 2010; Liu, 2015).
Aeromonas -associated diarrhea has been encountered worldwide in healthy persons across all age groups (Janda and Abbot, 2010; Percival and Williams, 2014a; Teunis and Figueras, 2016). Still, given that Aeromonas spp. are widely encountered in food and water, illness is observed in relatively few individuals who are exposed to the bacteria (Janda and Abbott, 2010). Gastrointestinal infections are more prevalent in developing countries (Ghenghesh et al., 2008). Susceptible groups include infants, young children, the elderly and persons with lowered immune status or underlying disease such as liver disease and malignant illnesses (Ghenghesh et al., 2008; Liu, 2015). Skin and soft tissue infections are often the result of trauma or penetrating injury and occur more frequently in adults than children (Janda and Abbot, 2010). For Aeromonas-associated bacteremia, the vast majority of cases are in immunocompromised individuals (Janda and Abbot, 2010). Antibiotics can be prescribed in severe cases where there is increased risk of infection spread (Percival and Williams, 2014a; Liu et al., 2015). PHAC has categorized drug-resistant Aeromonas spp. as a low priority for research and surveillance compared to other antimicrobial resistant pathogens (Garner et al., 2015). No human vaccines are currently available for Aeromonas infections (Liu et al., 2015).
Aeromonas –associated infections are not reportable illnesses in North America or in most countries worldwide. Case reports and outbreaks of illness have mostly been tied to food, hospital exposures, travel, non-water environments or unknown causes (Teunis and Figueras, 2016). Infections are more frequently observed during the warmer months (Janda and Abbot, 2010; Bhowmick and Battacharjee, 2018).
B.2.1.1.3 Sources and exposure
Aeromonas spp. can exist in virtually every ecosystem niche, including aquatic habitats, soils, vertebrate and invertebrate animal species, insects and foods (Janda and Abbot, 2010; Percival and Williams, 2014a). They are found in water and aquatic environments (e.g., lakes, rivers, groundwater, seawater, potable water supplies, wastewater and sewage) in all but the most extreme conditions of pH, temperature and salinity (Janda and Abbot, 2010). Members of the genus are found in the gastrointestinal tract of cold-blooded and warm-blooded animals including fish, birds, reptiles and domestic livestock. Aeromonas spp. can be isolated from the feces of healthy humans as a result of consumption of food and water containing the microorganisms (Percival and Williams, 2014a). They can be found in high concentrations in wastewater (Janda and Abbott, 2010; Percival and Williams, 2014a). Aeromonads grow optimally at elevated temperatures, thus concentrations in water are at their highest during the warmer months (LeChevallier et al., 1982; Gavriel et al., 2008; Chauret et al., 2001; Egorov et al., 2011).
Ingestion of contaminated food and water are considered the main routes of transmission for Aeromonas-associated gastroenteritis. Direct body contact with contaminated water is the primary method of transmission for Aeromonas spp. in water-related skin and soft tissue infections. Contaminated floodwaters in natural disaster settings have been identified as an important vehicle for these types of illnesses (Tempark et al., 2013). Person-to-person transfer is not considered a risk with Aeromonas infections.
Aeromonads are not commonly detected in the bulk water in municipal distribution systems with a disinfectant residual (Chauret et al., 2001; Egorov et al., 2011). In a survey of 293 public water systems in the U.S., Aeromonas spp. were detected by culture methods at 42 systems (14.3%), with concentrations ranging from 0.2 to 880 (median 1.6) CFU per 100 mL (Egorov et al., 2011). Groundwater is expected to have lower numbers of Aeromonads than surface waters, but drinking water wells can become colonized by the bacteria (Borchardt et al., 2003; Percival and Williams, 2014a; Katz et al. 2015). Aeromonads are capable of growth and persistence in distribution system biofilms and this can contribute to an increased recovery of the microorganisms from drinking water supplies (Gavriel et al., 1998; Chauret et al., 2001).
The importance of drinking water as a route of transmission for Aeromonas-related gastrointestinal illness is not clearly understood. Species of Aeromonas possessing multiple virulence genes have been detected in drinking water supplies in North America and in other countries (Handfield et al., 1996; Kühn et al., 1997; Sen and Rogers, 2004; Robertson et al., 2014b). Some investigations attempting to link strains recovered from drinking water supplies to patient isolates have been unsuccessful (Havelaar et al., 1992; Borchardt et al., 2003). Other studies have presented evidence of an epidemiological link between Aeromonas in clinical samples and drinking water as a source of infection (Khajanchi et al., 2010; Katz et al., 2015). It is generally accepted that only a subset of Aeromonas strains can cause gastrointestinal illness in humans (Teunis and Figueras, 2016). Furthermore, it is believed that infection is a complex process involving the virulence of the Aeromonas strain, its interaction with other microbes present in the gut (as co-infecting pathogens or as part of the natural microbiota) and the health status of the host (Teunis and Figueras, 2016). As a result, the presence of Aeromonas spp. in drinking water on its own is not sufficient to signify that a health risk exists (Edberg et al., 2007). More work is needed to determine the specific combination of host, environment and pathogen factors that lead to the occurrence of gastrointestinal illness associated with Aeromonas infections (Teunis and Figueras, 2016). No known drinking water outbreaks associated with Aeromonas have been recorded (Janda and Abbot, 2010; Teunis and Figueras, 2016).
B.2.1.1.4 Analytical methods
Standard methods for the detection of Aeromonas in drinking water are available (US EPA, 2001; APHA et al., 2017). However, there is no established universally-accepted culture-based method capable of detecting all Aeromonads in water samples (APHA et al., 2017). Aeromonas spp. are heterotrophic bacteria and are detected by heterotrophic plate count (HPC) tests; however, no direct correlation between HPC counts and Aeromonas concentrations exists.
B.2.1.1.5 Treatment considerations
When properly designed and operated, physical removal—chemically-assisted, slow sand, diatomaceous earth and membrane filtration or an alternative proven technology—and primary disinfection methods—chlorine, chlorine dioxide, ozone and UV— commonly used in drinking water treatment are very effective in reducing or inactivating Aeromonas spp. (Chauret et al., 2001; WHO, 2002; US EPA, 2006a; Yu et al., 2008). Monochloramine should not be used for primary disinfection due to its low oxidation potential; monochloramine is recommended only for secondary disinfection (i.e., to maintain a disinfectant residual in the distribution system) (Health Canada, 2019b). The use of granulated activated carbon (GAC) in water treatment may provide nutrient sources for aeromonads which can contribute to their presence and survival in drinking water distribution systems (WHO, 2002; US EPA, 2006a).
Aeromonas are as sensitive to chemical disinfectants as E. coli and other waterborne bacteria (Knøchel, 1991; Medema et al., 1991; Sisti et al., 1998; WHO, 2002; US EPA, 2006a). The CT requirements for inactivation of Aeromonas spp. by chemical disinfectants are less than those required for numerous enteric viruses. The UV dose requirements are comparable to other waterborne enteric bacterial pathogens and the enteric protozoa Giardia and Cryptosporidium and are less than those needed for many enteric viruses (Massa et al., 1999; Gerba et al., 2003; US EPA, 2006a; Health Canada, 2019b, 2019c).
General operational and maintenance practises for managing microbial survival and growth in drinking water distribution and plumbing systems as outlined in Part A are important for the control of Aeromonasspp. (Chauret et al., 2001; WHO, 2002; Percival and Williams, 2014a).
For residential-scale systems and private wells, regular physical inspection to identify deficiencies and testing of the water system (e.g., for E. coli and total coliforms) to confirm the microbiological quality of the water, are important. Where problems with the microbiological quality of the drinking water are suspected, it may be useful to include additional parameters (e.g., HPC) in the analysis (WHO and OECD, 2003, Health Canada, 2020c). Specific guidance on construction, operation, maintenance and testing should be obtained from the responsible drinking water authority in the affected jurisdiction.
B.2.1.1.6 International considerations
No guideline for Aeromonas spp. in drinking water has been established by the WHO, the EU, the US EPA or the Australian National Health and Medical Research Council (NHMRC, NRMMC 2011; WHO, 2017a; European Commission, 2020; US EPA, 2021a). In the Netherlands, Dutch drinking water legislation specifies a monitoring requirement for Aeromonas as an operational parameter with a target limit of < 1000 CFU/100 mL (Smeets et al., 2009). This target is based on treatment achievability and not on public health significance (WHO, 2002).
B.2.1.2 Legionella spp.
B.2.1.2.1 Description
The bacterial genus Legionella (Class: Gammaproteobacteria) comprises 61 species and 3 subspecies (LPSN, 2019). At least 30 species have been known to cause human infection (Cuhna et al., 2016; Burillo et al., 2017). Pathogenic Legionella spp. are opportunistic pathogens that cause respiratory illness in two main forms: Legionnaires’ disease and Pontiac fever (Percival and Williams, 2014e, NASEM, 2020). Legionella have also been associated with extrapulmonary infections, although these are much rarer (NASEM, 2020, CDC, 2021a). Illnesses caused by Legionella spp. are collectively known as legionellosis. Legionella pneumophila (mainly serogroup 1) is the most common and virulent pathogen of the genus, responsible for 65-90% of all cases of Legionnaires’ disease (Fields et al., 2002; Edelstein and Roy, 2015; Percival and Williams, 2014e, Prussin II et al., 2017). Diagnostic clinical tests are optimized for the detection of this species and serogroup, thus the proportion of disease caused by non-pneumophila, non-serogroup 1Legionella is likely underestimated (NASEM, 2020). OtherLegionella species can lead to disease, including:L. micdadei, L. bozmanae, L. dumoffii and L. longbeachae (Edelstein and Roy, 2015; Percival and Williams, 2014e; Cuhna et al., 2016).
The bacteria are Gram-negative, obligately aerobic, predominantly motile, short rod-shaped cells that require specific nutrients (L-cystine and iron) for growth (Percival and Williams, 2014e). During its life cycle, Legionella can adapt to fluctuating conditions by differentiating into cell types that vary in their infectivity and resistance to disinfection (Robertson et al., 2014a; NASEM, 2020).
B.2.1.2.2 Health effects
Legionnaires’ disease is a severe respiratory illness involving pneumonia, with symptoms that include fever, cough, chills, neurological aspects (confusion), muscle pain, headache and gastrointestinal problems (diarrhea, nausea, vomiting) (Castillo et al., 2016, Cunha et al., 2016; Edelstein and Roy, 2015). Symptom onset generally occurs 2 to 14 days after becoming infected (NASEM, 2020), and the disease can persist for weeks to several months (Palusińska-Szysz and Cendrowska-Pinkosz, 2009). Although many individuals are exposed to Legionella bacteria, few develop illness (Castillo et al., 2016). Legionnaires’ disease has a low attack rate, affecting less than 1-5% of the general population and less than 1-14% of hospital patients who are exposed to the bacteria during outbreaks (Hornei et al., 2007; Edelstein and Roy, 2015; Leoni et al., 2018). Legionnaires’ disease is more likely to occur in older adults or immunocompromised individuals, however healthy individuals can acquire Legionnaires’ disease if they are exposed to a high enough concentration of the bacteria (Springston and Yocavitch, 2017). Reports of Legionnaires' disease in healthy children are extremely rare (McDonough et al., 2007, Greenberg et al., 2006). Factors associated with an increased susceptibility to Legionnaires’ disease following exposure are male gender, age beginning at 40-50 years, smoking, chronic heart or lung disease, diabetes, chronic renal failure, immunosuppression, organ transplantation and some forms of cancer (Fields et al., 2002; Edelstein and Roy, 2015, Castillo et al., 2016; Cuhna et al., 2016; NASEM, 2020). The case fatality rate associated with Legionnaires’ disease depends on the underlying health of the patients, how quickly therapy is delivered and whether the cases are sporadic, hospital-acquired or outbreak-related (Edelstein and Roy, 2015). Mortality is estimated at less than 10-15% for community acquired cases, but can be higher than 25% for hospital acquired cases (Benin et al., 2002; Howden et al., 2003; Dominguez et al., 2009; Soda et al., 2017; Leoni et al., 2018).
Pontiac fever is a milder, flu-like, self-limiting and non-pneumonic disease associated with exposure to Legionella. The disease has mainly been diagnosed in outbreaks where individuals have flu-like symptoms and share exposure to aerosols from a common source (Lüttichau et al., 1998). How Pontiac fever develops is poorly understood and why some persons develop this disease while others develop Legionnaires’ disease is not known (Fields, et al., 2001; Edelstein, 2007). It has been proposed that Pontiac fever may be due to exposure to some combination of live and dead microorganisms (either Legionella species or coexisting microorganisms) and their products (including endotoxins) (Edelstein, 2007). Pontiac fever has a high attack rate, affecting as high as 80-90% of exposed individuals during outbreaks (Leoni et al., 2018). Symptoms appear from five hours to three days after infection and last for two to seven days. Long-term complications are not observed and the disease is not fatal (Tossa et al., 2006; Edelstein and Roy, 2015). There appear to be no predisposing host factors for Pontiac fever (Edelstein and Roy, 2015). Cases of Pontiac fever in children have been reported during outbreaks of the disease (Lüttichau et al., 1998; Goldberg et al., 1989, Jones et al., 2003; Burnsed et al., 2007).
Legionnaire’s disease and Pontiac fever are the most common manifestations of legionellosis; extrapulmonary disease caused by Legionella is extremely rare (NASEM, 2020, CDC, 2021a). Reported sites of extrapulmonary infections include the skin, joints and soft tissues which line the heart (Padrnos et al., 2014; Ibranosyan et al., 2019; CDC, 2021a). These infections have been most frequently observed in individuals who are immunosuppressed or who have concurrent Legionella pulmonary infections (Padrnos et al., 2014; Ibranosyan et al., 2019). Extrapulmonary infections are predominantly caused by Legionella species and strains other than L. pneumophila and L. pneumophila serogroup 1 (Padrnos et al., 2014; Ibranosyan et al., 2019).
Dose-response models have been developed for a few specific Legionella strains, derived from animal experiments (NASEM, 2020). No expert consensus exists on whether there is a threshold for detectable Legionella below which there is no risk of infection (NASEM, 2020).
Legionella is the major cause of waterborne illness outbreaks in the U.S. (Neil and Berkleman, 2008; CDC, 2017d; Friedman et al., 2017). Large Legionella outbreaks receive the most attention given their substantial health impact. However, it is estimated that less than 20% of all reported legionellosis cases are outbreak-related (Fields et al., 2002; Neil and Berkleman, 2008; Burillo et al., 2017). In Canada, reported rates of legionellosis in 2006-2016 (the latest year for which data have been published) were 0.37-1.39 (median: 0.71) per 100,000 population (PHAC, 2019b). Reported rates from the U.S. were 1.0-1.89 (median 1.18) per 100,000 population over the same period (Adams et al., 2016, 2017). As legionellosis is underdiagnosed and underreported, the actual number of cases is expected to be much higher (Castillo et al., 2016; PHAC, 2018d). A 22-month, multicentre Canada-wide study reported that 3.2% of patients diagnosed with community-acquired pneumonia (28/850) had Legionnaires’ disease (Marrie et al., 2003). Another Canadian study examining the frequency of Legionnaires’ disease in the summer months (May to October) found that 28% (9/33) of patients diagnosed with pneumonia tested positive for Legionella (Spiegelman et al., 2020). Legionellosis follows a distinct seasonal pattern, with the peak number of cases occurring during summer and fall (Prussin II et al., 2017, Cuhna et al., 2016). The yearly incidences of legionellosis in Canada and the U.S. are on the rise (Adams et al., 2016, 2017; PHAC, 2019b). Factors contributing to the yearly incidence rate include a true increase in the number of cases, greater use of diagnostic testing, and increased reporting (Burillo et al., 2017).
As Legionella are intracellular pathogens, treating Legionnaires’ disease requires the use of antibiotic agents capable of reaching therapeutic concentrations within human cells (Fields et al., 2002; Edelstein and Roy, 2015, Castillo et al., 2016; Wilson et al., 2018). No human vaccine for the disease exists (Edelstein and Roy, 2015). Most individuals with Pontiac fever do not become ill enough to seek medical attention, and antibiotic treatment is generally not required (Edelstein and Roy, 2015, Castillo et al., 2016). Trends in antibiotic resistance in Legionella spp. are not well understood (Wilson et al., 2018). Data on resistance of clinical isolates to antibiotics is not well documented due to the absence of easily performed tests (Wilson et al., 2018).
B.2.1.2.3 Sources and exposure
Legionella has two habitats—a primary reservoir in the natural environment and a secondary habitat in engineered water systems (NASEM, 2020). Its growth in these habitats is predominantly within free-living protozoa that reside within biofilms (Devos et al., 2005; NASEM, 2020). Legionella has been detected in freshwater and soil environments including lakes, rivers, sediments and groundwater worldwide (Fields et al., 2002; Percival and Williams, 2014e, Burillo et al., 2017; NASEM, 2020). Human and animal feces are not considered a source of Legionella, although it can be detected in the feces of infected individuals experiencing diarrhea symptoms. Animals can be infected by Legionella, but zoonotic transmission of the organism has not been documented (Surman-Lee et al., 2007; Edelstein and Roy, 2015).
As noted above, Legionella multiply inside protozoa (e.g., amoebae and ciliates) that are found in biofilms in natural waters and engineered water systems. Among them are: Acanthamoeba (see Section B.3.2.1), Hartmanella, Naegleria (see Section B.3.2.2), Valkampfia, Vermamoeba(formerly Hartmanella), Echinamoeba and Tetrahymena (Fields et al., 2002; Lau and Ashbolt, 2009; Buse et al., 2012, Percival and Williams, 2014e; NASEM, 2020). Survival within these protozoa provides a source of nutrients, a protective environment against disinfectants and other adverse conditions (such as elevated temperatures), and a means of transport (Percival and Williams, 2014e, Buse et al., 2012; NASEM, 2020). Legionella are also capable of persisting in biofilms in the absence of host protozoa (NASEM, 2020).
Legionella can be found in engineered water systems and equipment that support biofilm growth, including drinking water distribution systems, building and residential plumbing systems and cooling towers (NASEM, 2020). Low levels of Legionella can pass through treatment barriers, and grow in distribution system biofilms, where conditions are favourable. While Legionella is rarely detected in treated drinking water as it leaves the treatment plant (King et al., 2016; Hull et al., 2017), it is occasionally detected in drinking water distribution systems (Brooks et al., 2004; Pryor et al., 2004; Wang et al., 2012a; Lu et al., 2016; Hull et al., 2017; Waak et al., 2018; Dias et al., 2019; LeChevallier, 2019a,b). However, municipal drinking water distribution systems are not thought to be a major reservoir of Legionella (NASEM, 2020). It is important to note that storage facility sediments are known to harbour opportunistic pathogens, including Legionella (Lu et al., 2015; Qin et al., 2017). One legionellosis outbreak was attributed to a storage facility that had a low (<0.2 mg/L) free chlorine residual (Cohn et al., 2015).
Premise plumbing systems along with equipment supplied by these systems (e.g., cooling towers, hot tubs) are the priority area of concern for Legionella growth. Large complex premise plumbing systems, such as those found in hospitals, hotels, apartment buildings, community centres, industrial buildings and cruise ships, are significant sources of Legionella. Plumbing systems possess unique characteristics, which can promote the growth of Legionella to high concentrations. These characteristics include increased water temperatures, higher surface area to volume ratios, longer stagnation times, losses of disinfectant residual and the presence of nutrients. Legionella has been detected in both residential and non-residential buildings, in cold and hot water, at varying frequencies (Alary and Joly, 1991; Stout et al., 1992; Bates et al., 2000; Mathys et al., 2008; Donohue et al., 2014, 2019b; Bédard et al., 2015; Dilger et al., 2016; Collins et al., 2017; Hull et al., 2017; Dias et al., 2019; Gora et al., 2020). In general, the hot water supply system, because of its typically lower disinfectant residual concentrations and higher temperatures, tends to favour Legionella growth. Cold water supplies held at temperatures above 25°C can also have an increased risk of Legionella colonization (Donohue et al., 2014; Schwake et al. 2016).
Cooling towers or evaporative condensers in buildings and industry also represent a significant area of concern for Legionella growth. High rates of detection of Legionella, including L. pneumophila, have been reported for cooling towers (Llewellyn et al., 2017). Other potential sources of Legionella are car washes, decorative fountains and supermarket produce misters (NASEM, 2020).
Growth
Legionella typically grows at temperatures between 25 and 45°C, with an optimum temperature range between 25 and 35°C. Thus, water temperatures in this range support the highest levels of growth of this microorganism (NASEM, 2020). Legionella is also thermotolerant, meaning that it can survive at high temperatures, between 55 and 70°C (Allegra et al., 2008; Cervero-Aragó, 2015; 2019). Survival of Legionella in protozoan cysts following exposure to 80°C has been demonstrated (NASEM, 2020). Climate change and its associated temperature increases may facilitate Legionella growth (Cuhna and Cuhna, 2017; MacIntyre et al., 2018).
Transmission
Legionella has a water-to-air transmission, meaning inhalation of aerosols (size 2-10 µm) containing the bacteria is the main route of transmission (Percival and Williams, 2014e; Castillo et al., 2016). Generally, consumption of drinking water is not a recognized route of Legionella transmission (Percival and Williams, 2014e; Prussin II et al., 2017). It is hypothesized that microaspiration that occurs during drinking, or is associated with certain clinical conditions or procedures, is a potential source of exposure (NASEM, 2020). Inoculation of surgical wounds is another less common route of infection (Cuhna et al., 2016; Burillo et al., 2017). Person-to-person transmission generally does not occur (Percival and Williams, 2014e; Edelstein and Roy, 2015), but one probable case has been reported (Correia et al., 2016).
Given its route of transmission, fittings and equipment (e.g., showerheads, faucets, cooling towers, hot tubs, humidifiers and nebulizers, indoor fountains) capable of developing biofilms and of generating aerosols, represent potential sources of exposure to Legionella (NASEM, 2020).
Waterborne illness
Data on Legionella concentrations from epidemiological investigations and occurrence studies is limited, making it difficult to assess the extent of Legionella risk from various sources (NASEM, 2020). Epidemiological investigations of Legionnaires’ disease show that outbreaks are most commonly linked to building water systems, cooling towers and recreational facilities such as hot tubs (NASEM, 2020). The building categories most frequently involved are hotels and resorts, hospitals, long-term care facilities and industrial buildings (Walser et al., 2013; Garrison et al., 2016; Beauté, 2017). Notable outbreaks of Legionnaires’ disease in North America include Brooklyn, New York (2015: 138 cases, 16 deaths), Quincy, Illinois (2015: 58 cases, 12 deaths), Genessee County, Michigan (2014-2015: 87 cases, 12 deaths), Quebec City, Quebec (2012: 182 cases, 13 deaths) and Scarborough, Ontario (2005: 112 cases, 23 deaths) (Gilmour et al., 2007, Levesque et al., 2014, CDC, 2015a; MDHHS, 2016, Weiss et al., 2017). The collective understanding of the origins of sporadic cases of legionellosis is limited. Residential and non-residential building water systems appear to contribute to a substantial proportion of sporadic disease; and cooling towers are also a potentially significant source (Orkis et al., 2018). However, definitive linkage of cases to specific sources is difficult (Orkis et al., 2018). The importance of domestic water systems as a source of Legionella infection is not clear (Bates et al., 2000; Prussin II et al., 2017). Immunocompromised individuals are at greater risk for acquiring legionellosis from contaminated residential plumbing systems (NASEM, 2020).
Although legionellosis case rates are highest in the summer and fall, the report of a community-associated outbreak of Legionnaires’ disease in Calgary, Alberta in November and December of 2012 suggests that Legionella transmission can occur during the late fall and winter months in Canada (Knox et al., 2017). Rainfall and humidity have been associated with an increased risk of disease (Fisman et al., 2005; Beauté et al., 2016). Further changes in seasonality may be observed as result of climate change.
A comprehensive review and meta-analysis of Legionella occurrence data from outbreaks, sporadic cases and routine sampling programs at various water systems (cooling towers, wastewater treatment plants recreational facilities, buildings and residences) concluded that a Legionella concentration of 50,000 CFU/L warrants concern and should be considered an action level to trigger remedial activities). A lower action level may be necessary to protect individuals at higher risk for legionellosis, such as hospital patients (NASEM, 2020). Guidance material produced by numerous agencies vary in their recommendations for actions levels for Legionella or L. pneumophilain water, including for cooling towers (range: >1000 to >1,000,000 CFU/L) and potable water systems (range: >1000 to >10,000 CFU/L) (NASEM, 2020). More information is needed about environmental exposures that result in disease in order to inform health risk-based numerical values for Legionella in water.
B.2.1.2.4 Analytical methods
Standard methods for the detection of Legionella in drinking water are available (APHA et al., 2017; ISO 2019; AFNOR, 2021). Other methods may be approved for use in other jurisdictions. The literature can also be consulted for details on specific methods (Mercante and Winchell, 2015; Wang et al., 2017, Petricek and Hall, 2018). Building water systems vary substantially in their design, complexity and propensity for Legionella transmission. Thus environmental monitoring at individual facilities should be informed by a site-specific risk assessment as part of a Water Management Plan (HSE, 2013b; CDC, 2017a; ASHRAE, 2018). In general, monitoring programs consist of routine monitoring of general microbiological quality, as an indication of system control, in conjunction with testing for Legionella at regular time intervals (HSE 2013a; 2014; 2019; PWGSC, 2016). Historically, culture-based methods have been applied for monitoring (NASEM, 2020). Culture-based methods provide an acceptable measure of viability, but are time consuming and do not detect viable but non-culturable (VBNC) cells (Wang et al., 2017; NASEM, 2020). Quantitative PCR methods provide greater specificity and sensitivity and a shorter turnaround time when compared to culture-based methods; and they can detect VBNC cells (Wang et al., 2017; NASEM, 2020). A drawback of qPCR methods is that they capture all DNA, even from dead cells (NASEM, 2020). Both culture-based and qPCR methods can be applied for establishing baseline numbers of Legionella, flagging concerns and providing information indicative of growth, death or changes in the system (Wang et al., 2017, NASEM, 2020). Pulsed-field gel electrophoresis (PFGE) and sequence-based typing (SBT) are two common approaches to the molecular subtyping of Legionella (Raphael et al., 2016; Mercante and Winchell, 2015). For epidemiological investigations, sequence-based typing methods are the current gold standard for comparing environmental and patient isolates of Legionella (Gaia et al., 2005; Mercante and Winchell, 2015; APHA et al., 2017).
B.2.1.2.5 Treatment considerations
The general advice outlined in Part A is important for the control of Legionella spp. from source to tap (Falkinham et al., 2015b). The advice in Part A should be consulted along with the information provided below.
Treatment: When properly designed and operated, physical removal technologies—chemically-assisted, slow sand, diatomaceous earth and membrane filtration or an alternative proven technology—will reduce the number of Legionella present in drinking water (US EPA 1989, 2006b; Hijnen and Medema, 2010). Data from a 2019 study by the US EPA suggest that planktonic (i.e., freely floating) Legionella should be easily inactivated by free chlorine at CT values commonly applied during water treatment (see Appendix D). One study reported detection of Legionellaby qPCR at a facility that chloraminated the water (King et al., 2016). This detection serves as an important reminder that monochloramine should not be used for primary disinfection due to its low oxidation potential (Health Canada, 2019b). Biofilm-associated Legionella is much more resistant to disinfection (see distribution system section below). Research data indicates that UV dose requirements are greater than those needed for Giardia and Cryptosporidium, but are less than those needed for many enteric viruses (Hijnen et al., 2011; Health Canada, 2019b, 2019c). Providing effective control of free-living protozoa in drinking water (e.g., Acanthamoeba, Naegleria –see Section B.2.2) is also necessary for reducing Legionella populations (Loret and Greub, 2010; Thomas and Ashbolt, 2011; NASEM, 2020).
Drinking water distribution system: Buse et al. (2019) evaluated the protective effect that biofilms can have on the resistance of Legionella pneumophila to secondary disinfectants. CT values were determined for Legionella pneumophila strain Philadelphia-1 serogroup 1 when associated with biofilm on PVC material (see Table 3). As Table 3 demonstrates, free chlorine is a more powerful oxidant than monochloramine. However, water utilities typically maintain lower residuals with free chlorine. This has a significant impact on the amount of time required to inactivate Legionella when it is associated with biofilm as shown in Table 4. Thus, maintaining an effective disinfectant residual in the distribution system is essential to control the growth of Legionella.
Log inactivation | Free chlorine (mg∙min/L) | Monochloramine (mg∙min/L) |
---|---|---|
2 log | 8.86 | 17.16 |
3 log | 36.11 | 62.80 |
4 log | 63.67 | 108.44 |
Residual type | Residual concentration (mg/L) | Time required to achieve 2 log inactivation (minutes) |
---|---|---|
Free chlorine | 0.2 | 44.3 |
Free chlorine | 0.5 | 17.7 |
Free chlorine | 1.0Footnote a | 8.9 |
Monochloramine | 1.0 | 17.2 |
Monochloramine | 1.5 | 11.4 |
Monochloramine | 1.8Footnote a | 9.5 |
|
There is conflicting evidence with regards to whether free chlorine or monochloramine provides better control of Legionella (Donohue et al., 2019a; LeChevallier, 2019a,b). Monochloramine may trigger free-living amoeba trophozoites to form cysts which cannot host and support the growth of Legionella (Bukhari et al., 2018). However, the use of monochloramine may increase Mycobacterium spp. detections (Donohue et al., 2019a). Additional research is needed to determine optimized strategies for minimizing risks from opportunistic pathogens in drinking water distribution and premise plumbing systems (NASEM, 2020). Ideally, selected controls for Legionella should have benefits for the control of other pathogens in water systems (NASEM, 2020). The requirements for disinfectant residuals necessary to control Legionella in drinking water systems are under review by the US EPA (US EPA, 2021b).
The 2014-15 outbreaks of Legionnaires’ disease in Genesee County, Michigan which coincided with the Flint water crisis, provide an example of the unintentional consequences of changes in drinking water systems operations on distribution system water quality. A switch in the source of drinking water to Flint’s municipal system resulted in changes in water composition and distribution system conditions that ultimately resulted in a reduced free chlorine residual (Zahran et al., 2018). It is hypothesized that this disruption in water quality stimulated the growth of L. pneumophila in Flint’s distribution and plumbing systems and was responsible for the outbreaks (Zahran et al., 2018; Garner et al., 2019).
Premise plumbing: Many resources are available which address measures for reducing the risk of exposure to Legionella in building water systems. The National Building Code of Canada (NRCC, 2015a) and the National Plumbing Code of Canada (NPC) (NRCC 2015b) set out standards and technical provisions for the design and installation of HVAC systems and plumbing systems in buildings, respectively. Both contain provisions dealing with Legionella in building systems. The American National Standards Institute/American Society of Heating, Refrigerating, and Air-Conditioning Engineers (ANSI/ASHRAE) Standard 188 (ASHRAE, 2018) establishes minimum Legionella risk management requirements for building water systems intended for use by those involved in design, construction, installation, commissioning, operation, maintenance and service of centralized building water systems and components. Guidance documents recommend the use of water management/water safety plans for the management of Legionella in building water systems. Healthcare and long-term care facilities and buildings with cooling towers are identified as buildings with a particular need for water management programs to reduce the risk of Legionella growth and spread (WHO, 2007, HSE 2013a, CDC, 2017a). Publications to assist building managers in developing water management/water safety plans are available (WHO, 2007, 2011; HSE, 2013a, 2014; PWGSC, 2016; CDC, 2017a; ASHRAE, 2018). In general, the NASEM Committee on Management of Legionella in Water Systems recommends requirements for water management plans in all public buildings and establishing cooling tower registries as two policy initiatives that can improve public health protection from exposure to Legionella (NASEM, 2020).
The Province of Quebec enacted building safety legislation in 2013 which included regulations for the maintenance and operation of cooling towers (Government of Quebec, 2020). The regulations outline the requirements for owners that include registering their system with the regulator, implementing a water management plan and conducting regular testing for Legionella pneumophila. The city of Vancouver has also updated its building bylaws, requiring operational permits, maintainance logs and Legionella testing for cooling towers, evaporative condensers, decorative water features and rainwater harvesting and alternative water systems (City of Vancouver, 2021). Mandatory requirements for cooling tower registration have been in place in the City of Hamilton since 2011 (City of Hamilton, 2019).
For plumbing systems, temperature management, i.e., the use of control measures to keep the hot and cold water systems outside the microorganism’s growth range of 25-43°C, is a fundamental aspect of a Legionella control strategy (Bédard et al., 2016a; Boppe et al., 2016; NASEM, 2020). Maintaining a minimum hot water tank temperature of 60°C is a key threshold for reducing positive detection of Legionella in buildings (WHO, 2011; HSE, 2014; NRCC 2015b, NASEM, 2020). The NPC specifies that storing hot water at temperatures below 60°C in hot water tanks and delivery systems may lead to growth of Legionella bacteria. The NPC further specifies that electric storage-type water heaters should be pre-set to a temperature of 60°C as a result of the temperature stratification that can occur with this type of heater. Temperature stratification is not a concern for other types of water heaters with different designs that use different fuels (NRCC, 2015b). Adjusting temperature regimes to achieve temperature greater than 55°C at distal points in the system has also been recommended as an effective measure for reducing Legionella colonization (WHO, 2011; HSE, 2014, NASEM, 2020). The hot water temperatures required to prevent Legionella growth are associated with a higher scalding risk (NRCC 2015b, NASEM, 2020). Applications of temperature management strategies should operate in accordance with regulations in place regarding maximum allowable temperatures at the tap. The NPC specifies that water valves supplying showerheads and bathtubs should be capable of maintaining a water outlet temperature that does not exceed 49°C in order to reduce the risk of scalding (NRCC, 2015b). To meet temperature requirements, plumbing codes dictate the use of devices such as thermostatic mixing valves to ensure appropriate water temperatures (NASEM, 2020). These devices allow elevated water heater temperatures while protecting against scalding risk at the tap, yet can also provide surfaces for Legionella attachment and create favourable growth temperatures if located too far from taps and outlets (NASEM, 2020; Singh et al., 2020). The overall impact of these devices on control strategies is not clear (NASEM, 2020; Singh et al., 2020). Mixing valves should be positioned as close as possible to the point of use and provide access for maintenance and cleaning (WHO, 2007, NASEM, 2020). Temporarily elevating the water temperature, or heat shock (e.g., a stringent thermal shock of 70°C for 30 minutes), has been utilized as a control measure in building systems. However, the efficacy of this procedure is controversial, and it is considered an extreme remediation measure (NASEM, 2020).
Avoiding stagnation through proper system design and the use of flushing regimes is also essential for effective Legionella control (WHO, 2007, 2011; PWGSC, 2016; ASHRAE, 2018; NASEM, 2020). There is no consensus on the optimal flushing frequency required to mitigate Legionella risks (NASEM, 2020; Singh et al., 2020). Site assessments as part of a water management plan are recommended for informing and developing specific control strategies. Risks of stagnation in peripheral parts of plumbing systems can be minimized by regular use of outlets (HSE, 2014). Guidance documents for building systems recommend minimum weekly flushing of low flow pipe runs, dead ends/dead legs and infrequently used fittings or outlets (ECDC, 2017; HSE 2014, CDC, 2021b). For buildings with larger numbers of at-risk individuals, more frequent flushing may be needed, as determined by the risk assessment (WHO, 2007; HSE, 2014). Care should be taken during plumbing flushing procedures. Flushing can disturb biofilms and may generate contaminated aerosols containing Legionella (WHO, 2007; Singh et al., 2020). Resources for developing building flushing programs are available (Purdue University, 2020).
The use of on-site disinfection technologies can also be an important part of a Legionella control strategy in large building water systems (Bartram et al., 2007; US EPA, 2016; NASEM, 2020). Various disinfection technologies (free chlorine, monochloramine, chlorine dioxide, copper-silver ionization, UV light, ozone, point-of-use (POU)/point-of-entry (POE) filtration technologies) have demonstrated some level of effectiveness against Legionella (Bentham et al., 2007, Exner et al., 2007; US EPA, 2016; Springston and Yocavitch, 2017, NASEM, 2020). A water treatment professional should be consulted before applying any supplemental disinfection. Guidance materials on Legionella control in plumbing systems for health-care facilities have recommended minimum disinfectant residual targets of 0.3 mg/L (Moore and Shelton, 2004; WHO, 2007) to 0.5 mg/L (Australian Government, 2015) for free chlorine and 1.5 mg/L for monochloramine (Moore and Shelton, 2004). There is evidence that a monochloramine residual provides better control of Legionella in building water systems compared to free chlorine, although the reasons for the improved performance are not fully clear (NASEM, 2020).
The selection of any control strategy requires a detailed understanding of the complexity of the system and the composition of the water and system materials (Bartram et al., 2007; US EPA, 2016; NASEM, 2020). Advances in monitoring equipment (e.g., low cost sensors for temperature, oxidation-reduction potential) and data acquisition/analytics facilitates the implementation of real-time status and control of complex plumbing systems to identify and manage risks (Bédard et al., 2015; Saetta et al., 2021). “Smart” water meters can also provide useful data (e.g., water use, temperature, flow direction, pressure).
For homeowners, recommendations for the control of Legionella in household plumbing systems involve maintaining a minimum hot water tank temperature of 60°C, consistent with NPC specifications (NRCC 2015b; WHO, 2007; NASEM, 2020). Educating immunocompromised individuals on the potential risks from in-home equipment that create aerosols and may support Legionella growth (e.g., humidifiers, nebulizers) is a useful component of Legionellarisk management in the home (NASEM, 2020).
B.2.1.2.6 International considerations
The WHO, and the Australian National Health and Medical Research Council have not established a limit for Legionella in drinking water (WHO, 2017a; NHMRC, NRMMC 2011). The US EPA established a maximum contaminant level goal (MCLG, a non-enforceable guideline) of zero Legionella in drinking water in its 1989 Surface Water Treatment Rule (US EPA, 1989). The 2020 European Union Drinking Water Directive includes an action level of 1000 CFU/L for Legionella in premise plumbing systems (European Commission, 2020). Other guidelines or standards established for Legionella spp. in Canada, the U.S. and other countries worldwide relate to the operation and maintenance of premise plumbing in buildings.
B.2.1.3 Mycobacterium spp.
B.2.1.3.1 Description
The genus Mycobacterium (Class: Actinobacteria) contains over 200 recognized species. Bacteria belonging to this genus are diverse in their ability to cause disease in humans. Some are strict pathogens, whereas others cause opportunistic infections or are non-pathogenic. Tuberculosis and leprosy are two illnesses caused by Mycobacterium species; however, these particular species are not relevant to drinking water. The mycobacteria of concern for drinking water providers are the species collectively referred to as the non-tuberculous mycobacteria (NTM).
NTM are a group of over 150 distinct species that are considered to be opportunistic human pathogens (Falkinham, 2016a, 2016b). Members of the M. avium complex—which includes M. avium and its subspecies M. intracellulare and M. chimaera—are the microorganisms most frequently associated with human illness. Other medically-relevant species include M. abscessus,M. chelonae, M. fortuitum, M. gordonae, M. kansasii, M. malmoense and M. xenopi (Nichols et al., 2004; Hoefsloot et al., 2013; Falkinham, 2016a).
Mycobacteria are Gram-negative, aerobic to microaerophilic, non-motile, non-spore-forming rod-shaped bacteria. Species are categorized as either rapid growers or slow growers based on the time required to produce colonies on growth media (Cangelosi et al., 2004; Falkinham, 2015b). In general, mycobacteria grow at temperatures between15-45°C (George et al., 1980; Cangelosi et al., 2004). Optimal growth temperatures for individual species vary within the range of 30-45°C (De Groote, 2004a; Stinear et al., 2004). The bacteria are relatively heat-resistant, capable of surviving at temperatures greater than 50°C (Schulze-Robbecke and Buchholtz, 1992; Falkinham, 2016a). Mycobacteria can utilize many substances as nutrient sources and are able survive on very simple substrates (Kaur, 2014). All mycobacteria possess a thick and lipid-rich cell wall that makes the microorganisms relatively impermeable to hydrophilic compounds. This provides the bacteria with increased resistance to acid/alkaline conditions, disinfectants and antibiotics.
B.2.1.3.2 Health effects
NTM species cause a wide spectrum of diseases in humans (Whiley et al., 2012), even though disease in healthy humans is rarely reported. NTM infections occur largely in individuals who have weakened or suppressed immune status or in persons with underlying respiratory conditions. Risk factors for NTM diseases vary according to the type of disease.
Pulmonary disease is the most common form of NTM-associated illness (Griffith et al., 2007; Sharma and Upadhyay, 2020). There are two forms of NTM pulmonary disease. The first is the more traditional form, presenting with cavitary lesions and is seen in adults with underlying lung disease (De Groote, 2004b). Disease tends to occur more frequently in older males with a history of smoking, alcoholism or lung damage from occupational exposure to dusts and persons with chronic disease conditions that affect the lungs (e.g., cystic fibrosis, lung cancer, chronic obstructive pulmonary disease) (De Groote, 2004b; Falkinham, 2015c). The second form of disease presents as inflamed bronchi accompanied by nodules and is seen in individuals that lack classic risk factors or underlying disease (De Groote, 2004b). Disease tends to occur more frequently in older non-smoking females (De Groote, 2004b; Falkinham, 2015c). Features common to both diseases include persistent cough, weakness and night sweats (De Groote, 2004b; Falkinham, 2015c). The attack rates and time to onset of symptoms for pulmonary disease are not known. Infections can be difficult to diagnose from general respiratory illness and patients may have a long history of symptoms (e.g., months to years) before a diagnosis of mycobacterial disease is made (Falkinham et al., 2015b). Hypersensitivity pneumonitis, a form of pulmonary illness where inflammation within the lung is attributable to the body’s immune response to mycobacterial antigens, has also been associated with NTM-exposure (Whiley et al., 2012; Adjemian et al., 2018).
NTM may also cause disease involving extrapulmonary sites such as lymph nodes, skin and soft tissues, the bloodstream and other body sites (Whiley et al., 2012; Sharma and Upadhyay, 2020). Cervical lymphadenitis caused by NTM is a disease of childhood, marked by swollen lymph nodes in the head or neck (Bayazit et al., 2004; von Reyn et al., 2004). The majority of cases are seen in otherwise healthy children ranging in age from 18 months to 5 years (von Reyn et al., 2004, Falkinham, 2015c). It is suggested that erupting teeth may have a role in how this age group acquires the disease (Bayazit et al., 2004; Falkinham, 2015c). Specific risk factors for the disease in children remain unclear (von Reyn et al., 2004). Skin and soft tissue infections caused by NTM range from localized skin lesions or nodules to widespread ulcerative or necrotizing disease (Percival and Williams, 2014f). Gastrointestinal tract infections are common in individuals with acquired immunodeficiency syndrome (AIDS) (von Reyn et al., 2004). In immunodeficient individuals, NTM infections can spread to various parts of the body including joints, skin, blood, the liver and the brain (Percival and Williams, 2014f). NTM-associated bacteremia is a common and life-threatening infection in individuals with human immunodeficiency virus (HIV) or acquired immunodeficiency syndrome (AIDS) (Falkinham, 2015c). The NTM member M. avium subspecies paratuberculosis, is hypothesized to be a cause of Crohn’s disease, though evidence of an association is inconclusive (Waddell et al., 2015; 2016). Risk factors for non-pulmonary NTM diseases include other comorbidities that result in a compromised immunity, such as underlying immunological disorders and HIV infection; as well as injuries or procedures that can introduce infection through trauma (Adjemian et al., 2018). The infective doses for NTM species are not known (Stout et al., 2016; Hamilton et al., 2017; Adjemian et al., 2018). Mortality rates associated with cases of NTM illness are not well understood (Adjemian et al., 2018). In the U.S., the overall mortality burden associated with NTM disease has been estimated at 2.3 deaths per 1,000,000 person-years (Vinnard et al., 2016).
The incidence of illness associated with NTM in Canada is not known, as cases of illness are not reportable. In Ontario, the annual rates of pulmonary NTM disease were estimated at 9.7-10.7 cases per 100,000 population for the years 2006-2010 (Marras et al., 2013). In the U.S., NTM-associated infections are a reportable condition in only a small number of states (Donohue and Wymer, 2016; Adjemian et al., 2018). Donohue and Wymer (2016) reported that across five states (Maryland, Mississippi, Missouri, Ohio, and Wisconsin) the average annual rate of NTM cases of any type (pulmonary and extra-pulmonary), ranged from 8.7-13.9 cases per 100,000 population between 2008 and 2013. Population-based studies have shown that pulmonary cases can account for roughly 77–93% of the reported NTM cases (Cassidy et al., 2009; Donahue and Wymer, 2016). Data suggests the prevalence of pulmonary NTM disease is continually increasing in North America and worldwide (Marras et al., 2013; Donohue and Wymer, 2016; Stout et al., 2016, Adjemian et al., 2018). Few data are available on extra-pulmonary NTM disease prevalence in North America. In U.S. studies, estimates of the incidence of extra-pulmonary disease range from 1.5-1.9 cases per 100,000 population (Cassidy et al., 2009; Henkle et al., 2017, Adjemian et al., 2018). Little information is available on the impacts of seasonal- or climate-related factors on infections (Falkinham, 2004; Adjemian et al., 2018).
NTM are resistant to many commonly used antibiotics. Treatment typically requires a combination of antimicrobial antibiotics including clarithromycin, arithromycin, rifampin and others (Percival and Williams, 2014f; Falkinham, 2015c, Halstrom et al., 2015).
B.2.1.3.3 Sources and exposure
NTM are found in the environment in soil and in water habitats such as marine waters, lakes, rivers, streams, groundwater and swamps. Soils, in particular, acidic, peat-rich soils, are the primary reservoir (Falkinham, 2016b). Groundwater generally contains lower numbers of NTM than surface waters (Falkinham, 2015c). Wastewater and sewage sludge can contain high numbers of these microorganisms (Radomski et al., 2011; Percival and Williams, 2014f). Engineered water systems (e.g., drinking water distribution systems and building/premise plumbing) along with equipment supplied by plumbing systems (e.g., humidifiers, hot tubs, swimming pools) are important sources of NTM. These systems and equipment can provide nutrient, temperature and disinfection protection conditions that allow the bacteria to increase to high numbers. Mycobacteria are ideally suited to life in these environments because they are able to grow under low nutrient and oxygen conditions, are resistant to disinfectants, possess thermal tolerance, and can survive and grow in biofilms and free-living protozoa (Falkinham, 2015a).
Mycobacterium spp. have been frequently detected, by culture or qPCR, in treated drinking water; and at varying frequencies, in drinking water distribution systems (Le Dantec et al., 2002; Hilborn et al., 2006; Wang et al., 2012a; Thomson et al., 2013; Holinger et al., 2014; Whiley et al., 2014; Lu et al., 2016; King et al., 2016; Hull et al., 2017; Dias et al., 2019; Gora et al., 2020). The occurrence and concentrations of mycobacteria in municipal distribution systems using groundwater or surface water can be similar, despite typically different concentrations at the source (see above) (Covert et al., 1999; Lu et al., 2015). Sediments in drinking water storage facilities are also known to harbour Mycobacterium spp. (Lu et al., 2015; Qin et al., 2017).
Premise plumbing systems are a significant environment of concern for the growth of NTM. The microorganisms have been detected in both residential and non-residential buildings, in cold and hot tap water, at varying frequencies (Hilborn et al., 2006; Feazel et al., 2009; Wang et al., 2012a; Holinger et al., 2014; Dias et al., 2019; Donohue et al., 2019b; Lande et al., 2019; Gora et al., 2020). Higher rates of NTM contamination are found in buildings with recirculating hot water systems (e.g., hospitals, condominiums, apartment buildings) compared to private residences (Falkinham, 2015b; Li et al., 2017). NTM have been isolated from premise plumbing fittings and equipment supplied by plumbing systems including faucets, showerheads, hot tubs/spas, ice machines, swimming pools, footbaths and medical nebulizers (Percival and Williams, 2014f; Nichols et al., 2004). Gebert et al. (2018) reported that mycobacteria were far less abundant in showerheads from US homes receiving water from private wells as compared to those receiving municipal water.
Transmission
Inhalation of aerosols is the primary route of transmission for NTM pulmonary disease (De Groote, 2004b; Halstrom et al., 2015). Premise plumbing systems are the most credible sources for aerosol exposure (De Groote et al., 2004b; Halstrom et al., 2015). Soils and dust have also been identified as potential sources of aerosols (Adjemian et al., 2018; Halstrom et al., 2015). Ingestion of contaminated water is considered as a possible route of transmission that is relevant for gastrointestinal infections in HIV/AIDS patients (von Reyn et al., 2004; Corti and Palmero, 2008) and cervical lymphadenitis infections in children (Bayazit et al., 2004; Falkinham, 2015c). For NTM infections involving skin and soft-tissues and other body sites (e.g., blood, joints, bones, organs) transmission occurs through injuries or procedures (medical, cosmetic) that expose the site to contaminated water, soil or medical devices; or are a result of disseminated infections among immunocompromised individuals (De Groote and Johnson, 2004; von Reyn et al., 2004; Piersimoni and Scarparo, 2009; Falkinham, 2015b; Sharma and Upadhyay, 2020). Indirect person-to-person transfer (i.e., via contaminated objects) may be a relevant route of transmission for persons with cystic fibrosis (Bryant et al., 2013; Bryant et al., 2016, Sood and Parrish, 2017). M. avium ssp. paratuberculosis has a fecal-oral route of transmission in cattle; however, the pathogenic role of this organism in human disease and potential sources of infection are topics of considerable debate (Harris and Barrletta, 2001; Waddell et al., 2016). In general, animal-human transmission is not thought to be a significant route of exposure (Whiley et al., 2012; Falkinham 2015c).
Waterborne illness
It is difficult to identify the specific transmission routes and infection sources for NTM microorganisms. NTM are ubiquitous in water environments and the associated diseases have long incubation times (Nishiuchi et al., 2017; Adjemian et al., 2018). Verification of infection sources requires the identification of an identical genotype between clinical and environmental isolates (Halstrom et al., 2015; Nishiuchi et al., 2017; Ratnatunga et al., 2020). Infections with NTM are generally sporadic. Most outbreaks associated with NTM have been linked to treated recreational water facilities (swimming pools, hot tubs) or medical or cosmetic procedures (De Groote et al., 2004; De Groote and Johnson, 2004). No outbreaks of NTM disease have been linked to the consumption of drinking water in Canada or the U.S. (CDC, 2013 2015b; 2017d).
NTM are particularly problematic in healthcare facility water systems, causing a variety of diseases. Li et al. (2017) conducted a systematic review of NTM waterborne infections in health care facilities. Outbreaks of mycobacterial infection were found to occur in a range of settings including inpatient hospital units, outpatient procedure clinics, hemodialysis centers and operating rooms. Types of mycobacterial disease varied based on the route of exposure. Reported sites of infection included the bloodstream, respiratory tract, soft-tissues and other specific sites related to surgical procedures (Li et al., 2017). The most frequent routes of transmission were through exposure of catheters or surgical wounds to contaminated water supplies and exposures to non-sterile water during medical procedures (Li et al., 2017).
Residential plumbing systems have also been proposed as an important source of NTM exposure (Whiley et al., 2012; Halstrom et al., 2015). NTM pulmonary infections have been linked to exposure to aerosols from household water supplies and residential hot tubs (Halstrom et al., 2015). Skin infections from NTM associated with residential hot tub use have also been reported (Whiley et al., 2012). Other settings for case reports of extrapulmonary NTM infections tied to water uses have included: footbaths at a nail salon, home aquarium cleaning and tattoo parlours (Halstrom et al., 2015; Griffin et al., 2019). Monitoring results from premise plumbing systems in residential and non-residential buildings show that there are opportunities for human exposure to NTM but other factors (e.g., aerosols and host) are necessary for disease transmission (Donohue et al 2019b).
B.2.1.3.4 Analytical methods
Methods for isolation and culture-based detection of Mycobacterium spp. in drinking water have been described; however, there is presently no standardized approach to testing (APHA et al., 2017). Isolates can be identified to the genus and species level using PCR or DNA-sequencing methods (Stinear et al., 2004; Falkinham, 2015c). Identification to the subspecies or strain level requires more advanced molecular techniques (Stinear et al., 2004; Falkinham, 2015c). The literature can be consulted for details on specific methods (Stinear et al., 2004; Thomson et al., 2008; Wang et al., 2017).
B.2.1.3.5 Treatment considerations
The general advice outlined in Part A is important for the control of Mycobacterium spp. from source to tap (Falkinham et al., 2015a, 2015b). The advice in Part A should be consulted along with the information provided below.
Treatment: When properly designed and operated, physical removal technologies—chemically-assisted, slow sand, diatomaceous earth and membrane filtration or an alternative proven technology—are capable of reducing the number of mycobacteria in drinking water (LeChevallier et al., 2001; Le Dantec et al., 2002; LeChevallier, 2004). However, specific features of mycobacteria such as hydrophobicity and surface charge affect treatment processes in different ways (LeChevallier, 2004; Wong and Shin, 2015). Due to their highly hydrophobic cell wall, mycobacteria have an increased tendency to attach to particles (LeChevallier, 2004). Correlations between turbidity removal and removal of mycobacteria have been demonstrated (Falkinham et al., 2001; Wong and Shin, 2015). The use of GAC filters can provide conditions (accumulated nutrients, neutralized disinfectant residuals) which support the growth of mycobacteria (Le Dantec et al., 2002; LeChevallier, 2004).
Mycobacteria are very resistant to commonly used chemical disinfectants. Individual species and strains show significant variations in disinfectant sensitivity (Taylor et al., 2000; WHO, 2004); and reported CT values differ among investigators (WHO, 2004). The CT values reported by Taylor et al. (2000) for free chlorine (51-1552 mg∙min/L, see Appendix D) would be technically challenging to achieve at a treatment facility. Jacangelo et al. (2002) observed that the inactivation of Mycobacterium fortuitum required CT values for ozone equal to or greater than those required for Cryptosporidium.
The UV dose required to inactivate Mycobacterium avium complex microorganisms can be greater than those needed for Giardia and Cryptosporidiumand comparable to that required for some enteric viruses. It has been reported that the inactivation of some strains of M. avium and M. fortuitum require UV doses comparable to those required for adenovirus (Gerba et. al., 2003; Schiavano et al., 2018).
Even with effective treatment and disinfection in place, NTM have a strong tolerance for disinfection and can pass into distribution and plumbing systems in low numbers.
Drinking water distribution system: Full-scale studies suggest that free chlorine is more successful than monochloramine as a secondary disinfectant for controlling mycobacteria (Pryor et al., 2004; Wang et al., 2012a; Rhoads et al., 2017; Donohue et al., 2019b). Monochloramine may provide better control in biofilms on certain pipe materials such as corroded iron surfaces (Norton et al., 2004).
Premise plumbing: Guidance documents recommend the use of water management/water safety plans for the management of mycobacteria in building water systems (WHO, 2007). Resources are available to provide information for building managers (WHO, 2007; 2011). In health care facilities, control of mycobacteria will be achieved in part through management plans designed to reduce risk from Legionella (Ford et al., 2004). However, it should be recognized that mycobacteria and Legionella have differing sensitivity to drinking water disinfectants (Jacangelo et al., 2002, Pryor et al., 2004; Moore et al., 2006b).
Supplemental strategies described for control in hospital and health care facilities have included superheat and flush disinfection with hot water to temperatures above 50-70°C, the use of various disinfection strategies (free chlorine hyperchlorination, chlorine dioxide), and the use of POU membrane filtration technologies (LeChevallier, 2004; Sebakova et al., 2008; Williams et al., 2011; Hsu et al., 2016). Additional actions recommended as part of a water safety plan include regular cleaning and maintenance of plumbing fittings and equipment that can support biofilm growth and aerosol formation (faucets, showerheads, hot tubs/spas, cooling towers) (Ford et al., 2004).
For homeowners, maintaining hot water tank temperature consistent with NPC specifications for the control of Legionella (i.e., minimum of 60°C) (NRCC 2015b) has been recommended as part of a general strategy for minimizing the risks of exposure to opportunistic premise plumbing pathogens in the home (WHO, 2011; Falkinham et al., 2015a, 2015b; NASEM, 2020).
B.2.1.3.6 International considerations
No drinking water guideline for Mycobacterium spp. has been established by the WHO, the EU, the US EPA or the Australian National Health and Medical Research Council (NHMRC, NRMMC 2011; WHO, 2017a; European Commission, 2020; US EPA, 2021a).
B.2.1.4 Pseudomonas spp.
B.2.1.4.1 Description
The bacterial genus Pseudomonas (Class: Gammaproteobacteria) includes over 30 species (Chakravarty and Anderson, 2015). Pseudomonas aeruginosa is the most clinically relevant species and is an opportunistic pathogen capable of causing a variety of infections in humans (Chakravarty and Anderson, 2015; Daniels and Gregory, 2015). Other species (P. fluorescens, P. putida, P. stutzeri) have been infrequently reported in human infections (Chakravarty and Anderson, 2015).
Pseudomonas spp. are Gram-negative, strictly aerobic, motile, straight or slightly curved rod-shaped bacteria that grow over the range of 4-42°C (optimum: 28-37°C) (Moore et al., 2006a; Chakravarty and Anderson, 2015). They are metabolically versatile, capable of utilizing numerous substances as nutrient sources and surviving under low nutrient conditions (Chakravarty and Anderson, 2015; Falkinham et al., 2015a). Pseudomonas spp. are also significant due to their capacity to join or form biofilms in water environments (Bédard et al., 2016b).
B.2.1.4.2 Health effects
P. aeruginosa causes disease following colonization in patients where some predisposing factor (e.g., reduced immunity, underlying disease, traumatic injury or medical procedure) has made them more vulnerable to infection (Chakravarty and Anderson, 2015). The respiratory tract is the most common site of human infections. Symptoms typically include fever, chills, cough and laboured breathing; the onset can be sudden and severe (Daniels and Gregory, 2015). Cystic fibrosis patients are particularly prone to respiratory infection with P. aeruginosa, and the organism is a leading cause of morbidity and mortality in these individuals (Chakravarty and Anderson, 2015). P. aeruginosa is an important cause of infections involving the skin, eyes, ears and urinary tract (Chakravarty and Anderson, 2015; Daniels and Gregory, 2015). Bloodstream infections resulting from lung, skin or urinary tract infections can result in spread of the organism to other parts of the body. High mortality rates have been observed with P. aeruginosa septicaemia in high risk individuals (Chakravarty and Anderson, 2015; Daniels and Gregory, 2015). Individuals at higher risk for infections include those that have lowered immune status (e.g., patients with low neutrophil counts or HIV/AIDS); have underlying diseases (cystic fibrosis, diabetes, chronic pulmonary disease); are undergoing procedures with invasive medical devices (vascular and urinary catheters, ventilator, endotracheal tubes); or have breaches in host defenses as a result of burns or penetrating trauma (surgical incisions, wounds) (Daniels and Gregory, 2015). The doses of P. aeruginosa required to cause infection via the various transmission pathways are not well understood (Roser et al., 2014). P. aeruginosa infections in healthy individuals are rare.
Infections caused by Pseudomonas are not reportable illnesses in North America or in most countries worldwide. A review of outbreaks associated with water systems in healthcare settings identified the types of infection most commonly attributed to Pseudomonas as bloodstream, lung and urinary tract infections (Kanamori et al., 2016). Skin infections are the cause of the vast majority of Pseudomonas-associated outbreaks linked to recreational water venues that use treated water in the United States, with hotels the leading setting (Hlavsa et al., 2018).
Treatment of P. aeruginosa infections is difficult as a result of increasing antibiotic resistance (Falkinham et al., 2015a). Some strains have been found to be resistant to nearly all or all antibiotics including later generation beta-lactam antibiotics, fluoroquinolones and carbapenems (CDC, 2019a). Multidrug-resistant P. aeruginosa has been categorized as a “Serious Threat” by the CDC, and a priority for risk management attention by PHAC (CDC, 2019a; Garner et al., 2015). Carbapenem-resistant P. aeruginosa in particular have been identified by the WHO as a critical priority for developing new antibiotic strategies (WHO, 2017b).
B.2.1.4.3 Sources and exposure
Pseudomonas spp. are ubiquitous bacteria, found in a wide variety of habitats including soil, aquatic environments (fresh and marine surface waters, groundwater, potable water supplies) and vegetation (Falkinham et al., 2015a; Degnan, 2006). Human and animal feces are not a significant source; however, the microorganisms can be found in large numbers in sewage and wastewater (Degnan, 2006). Premise plumbing and equipment supplied by these systems that can provide the proper conditions for growth (e.g., nutrients, temperature, protection from disinfectants) are habitats for P. aeruginosa (Bédard et al., 2016b). Water supply systems in hospitals and other health-care settings are important sources of P. aeruginosa (Bédard et al., 2016b). Confirmed reservoirs in these settings include potable water faucets, sink and shower drains, humidifiers, water baths, hydrotherapy pools and bathing basins (Falkinham, 2015a; Bédard et al., 2016b). In community settings, hot tubs/spas and swimming pools can also be important sources of infections (Bédard et al., 2016b).
P. aeruginosa can be transmitted through person-to-person contact or through direct contact with contaminated objects or water (Falkinham, 2015a; Bédard et al., 2016b). Consumption of drinking water is not a recognized route of infection (Bédard et al., 2016b).
Studies involving both culture-based and molecular detection methods have found that P. aeruginosa is sporadically detected in treated water as it leaves the treatment plant and water and sediment samples from drinking water distribution systems (Wingender and Flemming, 2004; Van der Wielen and van der Kooij, 2013; Lu et al., 2015, 2016; Dias et al., 2019); and can be more frequently detected in samples collected from premise plumbing systems (Reuter et al., 2002; Rogues et al., 2007; Lavenir et al., 2008; Van der Wielen and van der Kooij, 2013; Charron et al., 2015). The amplification of P. aeruginosa populations within biofilms in premise plumbing or plumbing fittings is proposed as the reason for the increased detection in these samples (Bédard et al., 2016b). Within biofilms, Pseudomonas can survive and grow following ingestion by free-living amoebae such as Acanthamoeba spp. (see Section B.3.2.1) (Thomas and Ashbolt, 2011; Bédard et al., 2016b). The protective environment and nutrients provided by this interaction can contribute to the enhanced survival and dispersal of P. aeruginosa in drinking water distribution and premise-plumbing systems (Thomas and Ashbolt, 2011). No known drinking water outbreaks associated with P. aeruginosa have been recorded (CDC, 2004, 2006, 2008, 2011, 2013, 2015b, 2017d).
B.2.1.4.4 Analytical methods
Standard methods for the detection of Pseudomonas spp. in drinking water are available (APHA et al., 2017; ISO, 2019). The literature can also be consulted for details on specific methods (Wang et al., 2017). Pseudomonas spp. are heterotrophic bacteria and are detected by HPC tests; however, no direct correlation between HPC counts and P. aeruginosa concentrations exists.
B.2.1.4.5 Treatment considerations
The general advice outlined in Part A is important for the control of Pseudomonas spp. from source to tap (Falkinham et al., 2015a; Bédard et al., 2016b). The advice in Part A should be consulted along with the information provided below.
Treatment: When properly designed and operated, physical removal technologies—chemically-assisted, slow sand, diatomaceous earth and membrane filtration or an alternative proven technology—and primary disinfection methods—chlorine, chlorine dioxide, ozone and UV—commonly used in drinking water treatment are effective at removing or inactivating P. aeruginosa (LeChevallier and Au., 2004; Clauβ, 2006; Xue et al., 2013; Behnke and Camper, 2012, Zuma et al., 2009; Garvey et al., 2014; Zhang et al., 2015). For the inactivation of P. aeruginosa, the CT requirements for chlorine are less than those required for the inactivation of many enteric viruses and the UV dose requirements are less than those required for the enteric protozoa Giardia and Cryptosporidium (Clauβ, 2006; Xue et al., 2013; Health Canada, 2019b, 2019c). Monochloramine should not be used for primary disinfection due to its low oxidation potential; monochloramine is recommended only for secondary disinfection (i.e., to maintain a residual disinfectant in the distribution system) (Health Canada, 2019b).
Drinking water distribution system: Resistance to chlorination will vary depending on the strain and the protective effects provided by biofilms (Bédard et al., 2016; Mao et al., 2018). Laboratory-scale and pilot-scale studies suggest that maintaining free chlorine residuals above 0.3 mg/L is useful for control of Pseudomonas spp. in bulk water (Wang et al., 2012b; Mao et al., 2018). Mao et al., (2018) highlighted that long-term, continuous exposure to an effective free chlorine residual is important in order to prevent regrowth of Pseudomonas and the selection of resistant strains. Further research on the effects of chlorine-based disinfectants on P. aeruginosa in premise plumbing water and biofilms is needed (Bédard et al., 2016).
Premise plumbing: Water management/water safety plans are recommended for the management of Pseudomonas aeruginosa in building water systems (WHO, 2011). Supplemental strategies described as control measures in hospital and health care facilities have included superheat and flush disinfection with hot water to temperatures above 50-70°C, and the use of POU membrane filtration technologies (Falkinham et al., 2015a; Bédard et al., 2016b).
For homeowners, no specific actions have been identified as necessary to reduce their risk of P. aeruginosa infections. However, homeowners can minimize their risk of exposure to opportunistic waterborne pathogens by maintaining the temperature of their hot water tank at a minimum of 60°C (WHO, 2011; Falkinham et al., 2015a, 2015b).
B.2.1.4.6 International considerations
No drinking water guideline for P. aeruginosahas been established by the WHO, the EU, the US EPA or the Australian National Health and Medical Research Council (NHMRC, NRMMC 2011; WHO, 2017a; European Commission, 2020; US EPA, 2021a). Guidelines or standards developed for Pseudomonas spp. in Canada and the U.S. and other countries worldwide relate to control of the organism in building water systems outside of municipal distribution system networks.
B.2.2 Protozoa
B.2.2.1 Acanthamoeba spp.
B.2.2.1.1 Description
Acanthamoeba spp. are free-living amoebae commonly found in soil and aquatic environments. They are opportunistic pathogens that can cause rare but severe human diseases affecting the eye, skin, lungs, brain and central nervous system (Visvesvara et al., 2007; Chalmers, 2014a). Species of Acanthamoeba were originally classified based on differences in life stage (e.g., cyst – see below) morphology; however, genotyping is currently used to classify members of the genus (Visvesvara et al., 2007; Juárez et al., 2018). Approximately 20 different genotypes of Acanthamoeba have been identified based on gene sequence differences (Juárez et al., 2018). Acanthamoeba genotype T4 is the predominant type encountered in cases of illness and in the environment; however, other genotypes have also been associated with disease (Chalmers, 2014a; Juárez et al., 2018). Acanthamoeba spp. are also significant due to their ability to act as hosts for certain pathogenic microorganisms within drinking water systems.
Acanthamoeba spp. have low nutrient requirements and grow over the range of 12-45°C (optimum 30°C) (Chalmers, 2014a). Their lifecycle consists of two stages: a feeding trophozoite (25-40 µm) and resistant cyst (10-30 µm) that can withstand temperatures of -20°C-56°C and provide resistance to desiccation and disinfection (Chalmers, 2014a; Juárez et al., 2018).
B.2.2.1.2 Health effects
Acanthamoeba infections are rare in the general population (Visvesvara et al., 2007; Juárez et al., 2018). Acanthamoeba keratitis (AK) is the most common form of illness (Juárez et al., 2018). Early symptoms of AK include blurred vision, intense pain and photosensitivity, usually in one eye (Chalmers, 2014a; Juárez et al., 2018). In advanced and severe cases, symptoms include ulceration, swelling, glaucoma, cataract and blindness (Juárez et al., 2018). AK has a slow onset, taking days to several weeks to develop after infection, and the disease has a slow but severe progression (Köhsler et al., 2016, Juárez et al., 2018). In developed countries, AK primarily occurs among individuals who wear contact lenses (Chalmers, 2014a). Persons at increased risk of exposure include those who use unsterile tap water to store, wash or disinfect contact lenses; and persons who swim, use hot tubs or showers while wearing contact lenses (Chalmers, 2014a; Juárez et al., 2018). In the minority of AK cases that are not associated with contact lenses, the infections are generally associated with ocular trauma or environmental contamination (Chalmers, 2014a).
Other expressions of Acanthamoeba-associated disease are disseminated infections originating in the skin or lungs that can spread to areas such as the kidneys and adrenal glands; and granulomatous amoebic encephalitis (GAE), a fatal disease which occurs when infection spreads to the brain and central nervous system (Visvesvara et al., 2007; Chalmers, 2014a). These are very rare forms of illness and primarily affect individuals who have weakened or suppressed immune status or underlying disease (e.g., persons with HIV/AIDS, cancer, diabetes, liver disease or who are undergoing chemotherapy or organ transplantation) (Visvesvara et al., 2007; Chalmers, 2014a; Guimaraes et al., 2016). The numbers of Acanthamoeba spp. necessary to cause infections are not known.
Despite the widespread occurrence of the organism in environmental waters, the number of cases of illness caused by Acanthamoeba spp. is low. The estimated incidence of AK in developed countries is one to 33 cases per million contact lens wearers (CDC, 2017b). Treatment of AK is difficult, as the cysts are resistant to most antimicrobials at concentrations tolerated by the human eye (Juárez et al., 2018). Prolonged treatment with a combination of drugs is needed (Visvesvara et al., 2007).
B.2.2.1.3 Sources and exposure
Acanthamoeba spp. are ubiquitous in soil and water worldwide, and are one of the most common free-living amoebae occurring in the environment (Visvesvara et al., 2007; Juárez et al., 2018). The amoebae have been isolated from an abundance of natural and man-made environments including soil, mud, fresh and brackish waters, swimming pools, hot tubs/spas, cooling towers, humidifiers, heating, ventilation and air conditioning equipment, drinking water and airborne dust (Visvesvara et al., 2007; Chalmers, 2014a).
The relative importance of water as a pathway for infection is unclear. The ubiquitous presence of Acanthamoeba in the environment makes it difficult to determine sources of infection. Drinking and inhalation of contaminated water are not considered routes of infection (Chalmers, 2014a). No outbreaks of AK as a result of exposure to drinking water have been reported in North America (Kilvington et al., 2004; Craun et al., 2010; Yoder et al., 2012b). Cases of AK have been associated with the use of nonsterile tap water in the preparation of contact-lens solutions (Visvesvara et al., 2007). Disseminated infections and GAE caused by Acanthamoeba spp. are not thought to be waterborne (Chalmers, 2014a).
Acanthamoeba spp. can be commonly detected in drinking water distribution systems in North America and internationally (Magnet et al., 2012; Lu et al., 2016; Qin et al., 2017). Multiplication of Acanthamoeba spp. can occur in biofilms and loose deposits in drinking water distribution and premise plumbing systems (Thomas and Ashbolt, 2011; Wang et al., 2012a; Qin et al., 2017). In the U.S., Acanthamoeba spp. have been detected by PCR in 40-63% of municipal storage tank sediments (Lu et al., 2015; Qin et al., 2017).
Acanthamoeba spp. may serve as hosts for pathogenic amoebae-resisting microorganisms, providing conditions (nutrients, protection from environmental stresses) critical for the survival, amplification and transport of these organisms (Thomas and Ashbolt, 2011). It has been proposed that passage in free-living protozoa increases the virulence of amoebae-resisting microorganisms (Visvesvara et al., 2007; Thomas and Ashbolt, 2011; Chalmers, 2014a). Pathogenic bacteria isolated fromAcanthamoeba spp. include Legionella pneumophila, Mycobacterium avium, Helicobacter pylori, Escherichia coli serotype O157, Listeria monocytogenes, Pseudomonas spp. and Vibrio cholerae (Visvesvara et al., 2007; Juárez et al., 2018). Acanthamoeba spp. are also able to harbour protozoa, fungi and viruses (Köhsler et al., 2016; Juárez et al., 2018). More research is needed to determine the implications of the interactions between free-living amoeba species and pathogenic amoeba-resisting microorganisms in drinking water (Thomas and Ashbolt, 2011).
B.2.2.1.4 Analytical methods
No standardized methods have been established for the detection and identification of Acanthamoeba spp. in drinking water. Procedures for the isolation of Acanthamoeba in water samples involve concentration by membrane filtration or centrifugation, plaque screening and identification using molecular methods (Chalmers, 2014a). The literature can be consulted for details on specific methods (Wang et al., 2017).
B.2.2.1.5 Treatment considerations
Acanthamoeba cysts are larger than Giardia cysts and Cryptosporidium oocysts (Chalmers, 2014a; Health Canada, 2019b); thus, physical removal mechanisms used during drinking water treatment are expected to remove these cysts. The cysts are very resistant to commonly used chemical disinfectants and UV (Loret et al., 2008; Hijnen et al., 2011). For the inactivation of cysts of Acanthamoeba spp., the CT value reported by Loret et al. (2008) for free chlorine (1300 mg∙min/L, see Appendix D) would be technically challenging to achieve at a treatment facility. The CT values for chlorine dioxide and ozone are greater than those reported for Giardia and Cryptosporidium (Loret et al., 2008). The UV dose requirements for the inactivation of cysts of Acanthamoeba spp. are similar to those required for adenovirus (Hijnen et al., 2011; Health Canada, 2019b).
The general operational and maintenance practises important for the control of waterborne pathogens, including Acanthamoeba spp., in drinking water distribution and plumbing systems are outlined in Part A (Chalmers, 2014a; Ashbolt, 2015). As part of a general facility water management plan, building system managers may implement regular cleaning and maintenance of plumbing fittings and equipment that can support biofilm growth and aerosol formation (e.g., faucets, showerheads, hot tubs/spas, cooling towers). Control of Acanthamoeba spp. may be particularly important in some specialised uses of water such as emergency eye-wash stations (Chalmers, 2014a).
No specific homeowner actions are necessary. However, homeowners can minimize risks of exposure to opportunistic waterborne microorganisms in the home by maintaining the temperature of their hot water tank at a minimum of 60°C (WHO, 2011). Individuals in the home who wear contact lenses should also follow guidance from their eye care providers on proper lens handling, cleaning and wear (CDC, 2017b).
B.2.2.1.6 International considerations
No drinking water guideline for Acanthamoeba spp. has been established by the WHO, the EU, the US EPA or the Australian National Health and Medical Research Council (NHMRC, NRMMC 2011; WHO, 2017a; European Commission, 2020; US EPA, 2021a).
B.2.2.2 Naegleria fowleri
B.2.2.2.1 Description
Naegleria fowleri is a pathogenic free‐living amoeba that causes primary amebic meningoencephalitis (PAM) in humans, a rare but almost always fatal disease. Over 40 species of Naegleria spp. have been identified. However, to date, only N. fowleri has proven to be pathogenic to humans (Marciano‐Cabral & Cabral et al. 2007; Yoder et al. 2010). Eight known N. fowleri genotypes have been found worldwide, and all are suspected to be pathogenic to humans (Bartrand et al., 2014; Chalmers, 2014b). N. fowleri are thermophilic, grow well at 25-40°C (optimum: 37°C) and can tolerate temperatures exceeding 50-60°C (Hallenbeck & Brenniman, 1989; Visvesvara et al., 2007; Zaongo et al., 2018). There are three distinct phases in the N. fowlerilife cycle: a feeding and infectious trophozoite stage, an intermediate flagellate stage, and a resistant cyst stage (Bartrand et al., 2014, Chalmers, 2014b). Cysts can survive for long periods at temperatures well below those optimal for growth and are considerably resistant to disinfectants (Bartrand et al., 2014).
B.2.2.2.2 Health effects
Symptoms of PAM are clinically similar to bacterial or viral meningitis, beginning with headache, fever, nausea and vomiting and then moving to stiff neck, altered mental status, occasional hallucinations, seizures and coma (Visvesvara et al., 2007; Chalmers, 2014b). Onset of symptoms occurs within one to seven days of exposure and the disease progresses rapidly, with death generally occurring within five days (Visvesvara et al., 2007; Chalmers, 2014b). PAM has an extremely high fatality rate (greater than 97%) (De Jonckheere, 2011; Capewell et al., 2015). Among documented cases in the U.S., there have been only five known survivors (Capewell et al., 2015). Infection occurs when water containing N. fowleri enters the nasal passages. The amoebae invade the mucous membranes and travel along the olfactory nerve to the brain where they consume nerve and blood cells, causing inflammation and cell damage leading to death (Chalmers, 2014b; Siddiqui et al., 2016). The dose of N. fowleri necessary to cause infection is not well understood (Bartrand et al., 2014).
PAM occurs in otherwise healthy individuals with exposure to warm, untreated or poorly disinfected water (Yoder et al., 2010). The disease primarily affects children and young adults with a history of exposure to warm, recreational freshwaters. Reasons for the age distribution are unclear, but it is proposed that these age groups more frequently engage in water activities (e.g., diving, head submersion) that increase the risk of water moving forcefully up the nose (Visvesvara et al., 2007; Yoder et al., 2010). Despite the widespread occurrence of N. fowleri in environmental waters, PAM occurs infrequently. As of 2011, only 235 cases of PAM had been reported globally, with the majority occurring in the U.S. (De Jonckheere, 2011). Cases most often occur during the hot summer months.
PAM is difficult to detect, with most cases progressing so rapidly that diagnosis is made following death (Chalmers, 2014b). Several drugs have demonstrated effectiveness against N. fowleri in the laboratory, but their effectiveness in clinical treatment remains unclear (Capewell et al., 2015; Siddiqui et al., 2016). Survival of infection has been demonstrated in two cases using a combination of antimicrobial agents and aggressive management of brain-swelling (CDC, 2019b). Continued testing of therapies is necessary (Capewell et al., 2015; Siddiqui et al., 2016). No human vaccines for PAM exists (Siddiqui et al., 2016).
B.2.2.2.3 Sources and exposure
N. fowleri are naturally found in warm freshwater environments and soils worldwide (Chalmers, 2014b). The microorganisms have been isolated from a wide variety of natural and human-made warm water sources, including lakes, rivers, ponds, hot springs, geothermal groundwater, water receiving thermal discharges from power plants or industrial facilities and poorly maintained swimming pools (Chalmers, 2014b, Bartrand et al., 2014). In the U.S., N. fowleri has been most commonly encountered in natural waters in southern-tier states. However, PAM cases have also been reported from more northern states in the U.S. including Kansas, Indiana and Minnesota (Yoder et al., 2010; 2012a; Cope and Ali, 2016). N. fowleri has been isolated from drinking water and premise plumbing supplies in Australia and in Arizona, Louisiana and Texas in the U.S. (Bartrand et al., 2014, Villegas, 2020). Increasing ambient temperatures as a result of climate change may increase the geographical range of N. fowleri (Bartrand et al., 2014; Chalmers, 2014b).
Transmission of N. fowleri occurs through the intranasal route. While PAM cases have been reported from several countries, the most complete data comes from the U.S. (Cope and Ali, 2016). In the United States, most infections have been associated with recreational activities (e.g., swimming, diving, jumping, underwater play) in warm, fresh recreational waters (Yoder et al., 2010). The majority of exposures have occurred at lakes and ponds; exposures at rivers or streams have been less frequently reported (Yoder et al., 2010). Cases of illness have also been documented where improperly maintained swimming pools were the probable sources of exposure (Yoder et al., 2010; Cope and Ali, 2016). In very rare cases, infections have been linked to contaminated drinking water supplies, through activities such as nasal cleansing, bathing or recreational water uses (Yoder et al., 2010, 2012a, Bartrand et al., 2014). Drinking contaminated water is not a route of infection.
There is very little data on the occurrence of N. fowleri in North American drinking water distribution and premise plumbing systems (Bartrand et al., 2014). N. fowleri is widespread in environmental reservoirs but at low numbers, unless the environment provides conditions for amplification such as optimal growth temperatures, availability of nutrients and the absence of a disinfectant residual (Bartrand et al., 2014). Drinking water systems vulnerable to N. fowleri contamination are those where the temperature of the water supply continually exceeds 25°C and where effective disinfectant residuals are not maintained (Bartrand et al., 2014). Long-term survival of the cysts at temperatures below optimal growth temperatures is possible, and N. fowleri has the ability to survive over winter in lakes in subtropical and temperate regions (Bartrand et al., 2014). Laboratory-scale and full-scale studies have demonstrated that N. fowleri can persist and grow in distribution system and premise plumbing biofilms (Bartrand et al., 2014).
N. fowleri can act as a reservoir for amoeba-resisting microorganisms (Thomas and Ashbolt, 2011; Bartrand et al., 2014). Naegleria species are regarded as a host for L. pneumophila and can provide conditions which permit the replication, protection and distribution of this pathogen in the environment (Thomas and Ashbolt, 2011; Bartrand et al., 2014; Siddiqui et al., 2016). Significant research is needed in order to understand the interactions between free-living amoeba species and pathogenic amoeba-resisting microorganisms in order to quantify any risk to human health (Thomas and Ashbolt, 2011). Only six of 132 U.S. cases of N. fowleri reported between 1962 and 2013 have resulted from exposures related to drinking water (Yoder et al. 2010; CDC, 2017c). Three of the cases were linked to nasal cleansing (Louisiana (2): 2011, U.S. Virgin Islands (1), 2012), two were related to bathing (Arizona, 2002), and one was related to tap water exposure on an outdoor play slide (Louisiana, 2013) (Yoder et al. 2010, 2012a; Bartrand et al., 2014). The two Louisiana cases represent the first time disinfected tap water has been implicated in N. fowleri infection in the U.S. (Yoder et al., 2012a). To date, there have been no known cases of PAM documented in Canada.
B.2.2.2.4 Analytical methods
The detection and identification of N. fowleri in drinking water requires highly specialized laboratories (Bartrand et al., 2014, Chalmers, 2014b). No standardized methods have been established. Procedures for the isolation of N. fowleri involve concentration (membrane filtration or centrifugation) or separation, plaque screening and identification using immunofluorescence or molecular assays (Bartrand et al., 2014; Chalmers, 2014b, Wang et al., 2017). The literature can be consulted for details on specific methods (Bartrand et al., 2017; Wang et al., 2017).
B.2.2.2.5 Treatment considerations
When properly designed and operated, physical removal technologies—chemically-assisted, slow sand, diatomaceous earth and membrane filtration or an alternative proven technology—commonly used in drinking water treatment are expected to remove N. fowleri. The cysts are very resistant to commonly used primary disinfectants—chlorine and UV. N. fowleri cysts are very similar in size to Giardia cysts (Chalmers, 2014b, Health Canada, 2019b). For the inactivation of cysts of Naegleria spp. the CT requirements for free chlorine are similar to those needed for Giardia, whereas the UV dose requirements are greater than those needed for enteric protozoa but less than those needed for adenovirus (Sarkar and Gerba, 2012; Goudot et al., 2014; Health Canada, 2019b, 2019c). Monochloramine should not be used for primary disinfection due to its low oxidation potential; monochloramine is recommended only for secondary disinfection (i.e., to maintain a disinfectant residual in the distribution system) (Health Canada, 2019b).
The general operational and maintenance practises important for the control of waterborne pathogens, including Naegleria spp., in drinking water distribution and plumbing systems are outlined in Part A (Bartrand et al., 2014). Maintaining a minimum free chlorine residual of 0.5 mg/L throughout the distribution system is recommended for the control of N. fowleri in vulnerable drinking water systems (NHMRC, NRMMC, 2011; Louisiana Department of Health and Hospitals, 2013; Bartrand et al., 2014). The suggested best practice for a chloramine residual of greater than 1.5 mg/L throughout the distribution system (Health Canada, 2020b) is sufficient for N. fowleri control (NHMRC, NRMMC, 2011).
N. fowleri is not an immediate risk for drinking water systems in Canada. However, individuals should ensure that they conduct nasal rinses using water that has been boiled and cooled, or distilled water.
B.2.2.2.6 International considerations
No drinking water guideline for N. fowleri has been established by the WHO, the EU, the US EPA or the Australian National Health and Medical Research Council (NHMRC, NRMMC 2011; WHO, 2017a; European Commission, 2020; US EPA, 2021a).
Part C. References
Adams, D.A., Thomas, K.R., Jajosky, R.A., Foster, L., Sharp, P., Onweh, D.H., Schley, A.W. and Anderson, W.J. (2016). Summary of notifiable infectious diseases and conditions—United States, 2014. MMWR Morb. Mortal. Wkly. Rep., 63(54): 1-152.
Adams, D.A., Thomas, K.R., Jajosky, R.A., Foster, L., Baroi, G., Sharp, P., Onweh, D.H., Schley, A.W. and Anderson, W.J. (2017). Summary of notifiable infectious diseases and conditions—United States, 2015. MMWR Morb. Mortal. Wkly. Rep., 64(53): 1-143.
Adjemian, J., Daniel-Wayman, S., Ricotta, E. and Prevots, D.R. (2018). Epidemiology of nontuberculous mycobacteriosis. Seminars in Respiratory and Critical Care Medicine, 39(3): 325-335.
AFNOR (2021). Water analysis. AFNOR Certification. Saint Denis La Plaine, France. Accessed July 2021 from: https://nf-validation.afnor.org/en/water-analysis/
Alary, M. and Joly, J.R. (1991). Risk factors for contamination of domestic hot water systems by Legionellae. Appl. Environ. Microbiol., 57(8): 2360-2367.
Allegra, S., Berger, F., Berthelot, P., Grattard, F., Pozzetto, B. and Riffard, S. (2008). Use of flow cytometry to monitor Legionella viability. Appl. Environ. Microbiol., 74 (24):7813-7816.
APHA, AWWA and WEF. (2017). Standard methods for the examination of water and wastewater. 23rd edition. American Public Health Association, American Water Works Association and Water Environment Federation, Washington, DC.
Ashbolt, N.J. (2015). Environmental (saprozoic) pathogens of engineered water systems: Understanding their ecology for risk assessment and management. Pathogens, 4(2): 390-405.
ASHRAE. (2018). ANSI/ASHRAE Standard 188-2018, Legionellosis: Risk Management for Building Water Systems. American Society of Heating, Refridgerating and Air Conditioning Engineers. Atlanta, GA. www.ashrae.org.
Australian Government (2015). Guidelines for Legionella control in the operation and maintenance of water distribution systems in health and aged care facilities. Australian Government, Canberra. Accessed July 2021 from: https://www.health.gov.au/
AWWA (2021). Climate action plans—Adaptive management strategies for utilities. Manual of water supply practices—M71. 1st edition. American Water Works Association, Denver, Colorado.
Backert, S., Tegtmeyer, N., Cróinín, T.Ó, Boehm, M. and Heimesaat, M.M. (2016). Human campylobacteriosis. In Campylobacter: Features, detection, and prevention of foodborne disease. Klein, G (ed.). Academic Press. Cambridge, USA. pp. 1-25.
Baker, K.H. and Hegarty, J.P. (2001). Presence of Helicobacter pylori in drinking water is associated with clinical infection (2001) Scand. J. Infect. Dis., 33 (10):744-746.
Baker, K.H., Hegarty, J.P., Redmond, B., Reed, N.A. and Herson, D.S. (2002). Effect of oxidizing disinfectants (chlorine, monochloramine, and ozone) on Helicobacter pylori. Appl. Environ. Microbiol., 68(2): 981-984.
Bartram, J., Bentham, R., Briand, E., Callan, P., Crespi, S., Lee, J.V. and Surman-Lee, S. (2007). Chapter 3 approaches to risk management. In Legionella and the prevention of legionellosis. Bartram, J. (ed.). World Health Organization, Geneva, Switzerland. pp. 39-56.
Bartrand, T.A., Causey, J.J. and Clancy, J.L. (2014). Naegleria fowleri: An emerging drinking water pathogen. J. Am. Water Works Assoc., 106(10): E418-E432.
Bates, M.N., Maas, E., Martin, T., Harte, D., Grubner, M. and Margolin, T. (2000). Investigation of the prevalence of Legionella species in domestic hot water systems. New Zealand Med. J., 113(1111): 218-220.
Bayazit, Y.A., Bayazit, N. and Namiduru, M. (2004). Mycobacterial cervical lymphadenitis. Orl, 66(5): 275-280.
Beauté, J., Sandin, S., Uldum, S.A., Rota, M.C., Brandsema, P., Giesecke, J. and Sparén, P. (2016). Short-term effects of atmospheric pressure, temperature, and rainfall on notification rate of community-acquired Legionnaires' disease in four European countries. Epidemiol. Infect., 144(16): 3483-3493.
Beauté J, on behalf of the European Legionnaires’ Disease Surveillance Network. Legionnaires’ disease in Europe, 2011 to 2015. Euro Surveill. 2017; 22(27):30566.
Bédard, E., Fey, S., Charron, D., Lalancette, C., Cantin, P., Dolcé, P., Laferrière, C., Déziel, E. and Prévost, M. (2015). Temperature diagnostic to identify high risk areas and optimize Legionella pneumophila surveillance in hot water distribution systems. Wat. Res., 71:244-256.
Bédard, E., Boppe, I., Kouamé, S., Martin, P., Pinsonneault, L., Valiquette, L., Racine, J. and Prévost, M. (2016a). Combination of heat shock and enhanced thermal regime to control the growth of a persistent Legionella pneumophila strain. Pathogens, 5(2): 35.
Bédard, E., Prévost, M. and Déziel, E. (2016b). Pseudomonas aeruginosa in premise plumbing of large buildings. MicrobiologyOpen, 5(6): 937-956.
Behnke, S. and Camper, A.K. (2012). Chlorine dioxide disinfection of single and dual species biofilms, Benin, A.L., Benson, R.F. and Besser, R.E. (2002). Trends in Legionnaires’ disease, 1980–1998: Declining mortality and new patterns of diagnosis. Clin. Infect. Dis., 35(9): 1039-1046.
Bentham, R., Surman-Lee, S., Lee, J.V., Briand, E. and Van de Kooj, D. (2007). Chapter 4: Potable water and in-building distribution systems. In Legionella and the prevention of legionellosis. Bartram, J. et al. (eds.). World Health Organization, Geneva, Switzerland. pp. 57-68.
Bernstein, C.N., McKeown, I., Embil, J.M., Blanchard, J.F., Dawood, M., Kabani, A., Kliewer, E., Smart, G., Coghlan, G., MacDonald, S., Cook, C., Orr, P. (1999). Seroprevalence of Helicobacter pylori, incidence of gastric cancer, and peptic ulcer-associated hospitalizations in a Canadian Indian population. Digest. Dis. Sci., 44 (4): 668-674.
Bhowmick, U.D. and Bhattacharjee, S. (2018). Bacteriological, clinical and virulence aspects of Aeromonas-associated diseases in humans. Pol. J. Microbiol., 67(2): 137-149.
Black, R.E., Levine, M.M., Clements, M.L., Highes, T.P. and Blaser, M.J. (1988). Experimental Campylobacter jejuni infection in humans. J. Infect. Dis. 157, 472-479.
Boppe, I., Bédard, E., Taillandier, C., Lecellier, D., Nantel-Gauvin, M. A., Villion, M., Laferrière, C. and Prevost, M. (2016). Investigative approach to improve hot water system hydraulics through temperature monitoring to reduce building environmental quality hazard associated to Legionella. Build. Environ., 108, 230-239.
Borchardt, M.A., Stemper, M.E. and Standridge, J.H. (2003). Aeromonas isolates from human diarrheic stool and groundwater compared by pulsed-field gel electrophoresis. Emerg. Infect. Dis., 9(2): 224-228.
Bragança, S.M., Azevedo, N.F., Simöes, L.C., Keevil, C.W. and Vieira, M.J. (2007). Use of fluorescent in situ hybridisation for the visualisation of Helicobacter pylori in real drinking water biofilms. Water Sci. Technol., 55(8-9):387-393.
Brennhovd, O., Kapperud, G. and Langeland, G. (1992). Survey of thermotolerant Campylobacter spp. and Yersinia spp. in three surface water sources in Norway. Int. J. Food Microbiol., 15(3-4): 327-338.
Brooks, T., Osicki, R.A., Springthorpe, S., Sattar, S.A., Filion, L. Abrial, D. and Riffard, S. (2004). Detection and identification of Legionella species from groundwaters. J. Toxicol. Environ. Health Part A, 67:1845–1859.
Brown, L.M. (2000). Helicobacter pylori: Epidemiology and routes of transmission. Epidemiol. Rev., 22 (2): 283-297.
Bryant, J.M. et al. (2016). Population-level genomics identifies the emergence and global spread of a human transmissible multidrug-resistant nontuberculous Mycobacterium. Science, 354(6313): 751-757.
Bryant, J.M., Grogono, D.M., Greaves, D., Foweraker, J., Roddick, I., Inns, T., Reacher, M., Haworth, C.S., Curran, M.D., Harris, S.R., Peacock, S.J., Parkhill, J. and Floto, R.A. (2013). Whole-genome sequencing to identify transmission of Mycobacterium abscessus between patients with cystic fibrosis: A retrospective cohort study. Lancet, 381(9877): 1551-1560.
Bukhari, Z., LeChevallier, M.W, Jjemba, P., Johnson, W., Haas, C. and Hamilton, K. (2018). Development of a risk management strategy for Legionellain reclaimed water systems. Project Reuse-12-05. Water Research Foundation, Denver, Colorado.
Burillo, A., Pedro-Botet, M.L. and Bouza, E. (2017). Microbiology and epidemiology of Legionnaires’ disease. Infect. Dis. Clin. North Am., 31(1): 7-27.
Burnsed, L.J., Hicks, L.A., Smithee, L.M.K., Fields, B.S., Bradley, K.K., Pascoe, N., Richards, S.M., Mallonee, S., Littrell, L., Benson, R.F. and Moore, M.R. (2007). A large, travel-associated outbreak of legionellosis among hotel guests: Utility of the urine antigen assay in confirming Pontiac fever. Clin Infect Dis, 44(2): 222-228.
Buse, H.Y., Schoen, M.E. and Ashbolt, N.J. (2012). Legionellae in engineered systems and use of quantitative microbial risk assessment to predict exposure. Water Res., 46 (4): 921-933.
Buse, H.Y., Morris, B.J., Struewing, I.T. and Szabo, J.G. (2019). Chlorine and monochloramine disinfection of Legionella pneumophila colonizing copper and polyvinyl chloride drinking water biofilms. Appl. Environ. Microbiol., 85(7): e02956-18.
Butler, A.J., Pintar, K.D.M. and Thomas, M.K. (2016). Estimating the Relative Role of Various Subcategories of Food, Water, and Animal Contact Transmission of 28 Enteric Diseases in Canada. Foodborne Pathog. Dis., 13 (2): 57-64.
Calero Preciado, C., Boxall, J., Soria-Carrasco, V., Martínez, S. and Douterelo, I. (2021). Implications of climate change: How does increased water temperature influence biofilm and water quality of chlorinated drinking water distribution systems? Front. Microbiol., 12.
Cangelosi, G., Clark-Curtiss, J., Behr, M., Bull, T. and Stinear, T. (2004). Biology of waterborne pathogenic mycobacteria. In Pathogenic mycobacteria in water—A guide to public health consequences, monitoring and management. IWA Publishing. World Health Organization. Geneva, Switzerland. pp. 39-54.
Cantor, A.F. (2017). Optimization of phosphorus-based corrosion control chemicals using a comprehensive perspective of water quality. Report #4586. Water Research Foundation, Denver, Colorado.
Capewell, L.G., Harris, A.M., Yoder, J.S., Cope, J.R., Eddy, B.A., Roy, S.L., Visvesvara, G.S., Fox, L.M. and Beach, M.J. (2015). Diagnosis, clinical course, and treatment of primary amoebic meningoencephalitis in the United States, 1937-2013. J. Pediatric Infect. Dis. Soc., 4(4): e68-e75.
Cassidy, P.M., Hedberg, K., Saulson, A., McNelly, E. and Winthrop, K.L. (2009). Nontuberculous mycobacterial disease prevalence and risk factors: A changing epidemiology. Clin. Infect. Dis. 49 (12):e124-e129.
Castillo, N.E., Rajasekaran, A. and Ali, S.K. (2016). Legionnaires' disease: A review. Infect. Dis. Clin. Pract., 24(5): 248-253.
CDC. (2004). Surveillance for Waterborne-Disease Outbreaks Associated with Recreational Water—United States, 2001–2002 and Surveillance for Waterborne Disease Outbreaks Associated with Drinking Water—United States, 2001–2002. In Surveillance Summaries, October 22, 2004. MMWR 2004:53(no. SS-8).
CDC. (2006). Surveillance for waterborne disease and outbreaks associated with drinking water and water not intended for drinking--United States, 2003-2004. MMWR Surveill Summ, 55(12): 31-65.
CDC. (2008). Surveillance for Waterborne Disease and Outbreaks Associated with Recreational Water use and Other Aquatic Facility-Associated Health Events—United States, 2005–2006 and Surveillance for Waterborne Disease and Outbreaks Associated with Drinking Water and Water Not Intended for Drinking—United States, 2005–2006. Surveillance Summaries. MMWR 2008;57(no. SS-9).
CDC. (2011). Surveillance for waterborne disease outbreaks associated with drinking water---United States, 2007--2008. MMWR Surveill. Summ., 60(12): 38-68.
CDC. (2013). Surveillance for Waterborne Disease Outbreaks Associated with Drinking
Water and Other Nonrecreational Water—United States, 2009–2010. Surveillance Summaries. MMWR 2013;62(no. 35).
CDC. (2015a). Epi-Aid 2015-037 Trip Report: “Undetermined source of an healthcare-associated outbreak of Legionnaire’s disease—Illinois, 2015”. Centres for Disease Control and Prevention, Atlanta, GA. Accessed July 2021 from: http://www.dph. illinois.gov/sites/default/files/publications/cdc-trip-reportquincyil12. 31.15.pdf.
CDC. (2015b). Surveillance for Waterborne Disease Outbreaks Associated with
Drinking Water—United States, 2011–2012. Surveillance Summaries. MMWR 2015;64 (no. 31).
CDC. (2017a). Developing a water management program to reduce Legionellagrowth & spread in buildings. A practical guide to implementing industry standards. Version 1.1. Centers for Disease Control and Prevention. Atlanta, GA. Accessed November 2019 from: https://www.cdc.gov/legionella/wmp/toolkit/index.html
CDC. (2017b). Parasites—Acanthamoeba—Granulomatous Amebic Encephalitis (GAE); Keratitis. Centers for Disease Control and Prevention. Atlanta, GA. Accessed July 2019 from: https://www.cdc.gov/parasites/acanthamoeba/index.html
CDC. (2017c). Parasites—Naegleria fowleri—Primary Amebic Meningoencephalitis (PAM)—Amebic Encephalitis. Naegleria fowleri in Louisiana Public Water Systems. Centers for Disease Control and Prevention. Atlanta, GA. Accessed July 2019 from: https://www.cdc.gov/parasites/naegleria/public-water-systems-louisiana.html
CDC. (2017d). Surveillance for waterborne disease outbreaks associated with drinking water -United States, 2013-2014. MMWR., 2017; 66(44): 1216-1221.
CDC. (2018). Centers for Disease Control and Prevention. National Notifiable Diseases Surveillance System, 2017 Annual Tables of Infectious Disease Data. Atlanta, GA. CDC Division of Health Informatics and Surveillance, 2018. Available at https://wonder.cdc.gov/nndss/nndss_annual_tables_menu.asp
CDC. (2019a). Antibiotic Resistance Threats in the United States, 2019. Atlanta, GA: U.S. Department of Health and Human Services, CDC; 2019. Accessed March 2022 from: https://www.cdc.gov/drugresistance/Biggest-Threats.html
CDC. (2019b). Parasites—Naegleria fowleri—Primary Amebic Meningoencephalitis (PAM)—Amebic Encephalitis. General Information. Centers for Disease Control and Prevention. Atlanta, GA. Accessed July 2019 from: https://www.cdc.gov/parasites/naegleria/general.html
CDC. (2021a). Legionella (Legionnaires' Disease and Pontiac Fever) - Clinical Features. Centers for Disease Control and Prevention. Atlanta, GA. Accessed June 2021 from: https://www.cdc.gov/legionella/clinicians/clinical-features.html
CDC. (2021b). Toolkit for controlling Legionella in common sources of exposure (Legionella Control Toolkit). Centers for Disease Control and Prevention. Atlanta, GA. Accessed May 2021 from: https://www.cdc.gov/legionella/wmp/control-toolkit/index.html
Cervero-Aragó, S., Rodríguez-Martínez, S., Puertas-Bennasar, A. and Araujo, R.M. (2015). Effect of common drinking water disinfectants, chlorine and heat, on free Legionella and amoebae-associated Legionella. PLoS ONE, 10 (8), art. no. e0134726.
Cervero-Aragó, S., Schrammel, B., Dietersdorfer, E., Sommer, R., Lück, C., Walochnik, J. and Kirschner, A. (2019). Viability and infectivity of viable but nonculturable Legionella pneumophila strains induced at high temperatures. Water Res., 158: 268-279.
Chakravarty, S. and Anderson, G.G. (2015). The genus Pseudomonas. In Practical handbook of microbiology. Goldman, E. and Green, L.H. (eds.). Third. CRC Press, Taylor & Francis Group, Boca Raton, pp. 321-343.
Chalmers, R.M. (2014a). Acanthamoeba. In Microbiology of waterborne diseases: Microbiological aspects and risks: Second edition. Elsevier Ltd. Oxford, UK pp. 263-276.
Chalmers, R.M. (2014b). Naegleria. In Microbiology of waterborne diseases: Microbiological aspects and risks: Second edition. Elsevier Ltd. Oxford, UK pp. 407-416.
Charron, D., Bédard, E., Lalancette, C., Laferrière, C. and Prévost, M. (2015). Impact of electronic faucets and water quality on the occurrence of Pseudomonas aeruginosa in water: A multi-hospital study. Infect. Control Hosp. Epidemiol., 36(3): 311-319.
Chauret, C., Volk, C., Creason, R., Jarosh, J., Robinson, J. and Warnes, C. (2001). Detection of Aeromonas hydrophila in a drinking-water distribution system: A field and pilot study. Can. J. Microbiol., 47(8): 782-786.
Chauret, C., Smith, C. and Baribeau, H. (2008). Inactivation of Nitrosomonas europaea and pathogenic Escherichia coli by chlorine and monochloramine. J. Water Health, 6(3): 315-322.
Cheyne, B.M., Van Dyke, M.I., Anderson, W.B. and Huck, P.M. (2009). An evaluation of methods for the isolation of Yersinia enterocolitica from surface waters in the grand river watershed. J. Water Health, 7(3): 392-403.
Cheyne, B.M., Van Dyke, M.I., Anderson, W.B. and Huck, P.M. (2010). The detection of Yersinia enterocolitica in surface water by quantitative PCR amplification of the ail and yadA genes. J. Water Health, 8(3): 487-499.
Christenson, J.C. (2013). Salmonella infections. Pediatr Rev, 34(9): 375-383.
City of Hamilton. (2019). Accessed February, 2020 from: https://www.hamilton.ca/operating-business/health-requirements-inspections/cooling-towers
City of Vancouver (2021). Get a water systems operating permit. Vancouver, Canada. Accessed July 2021 from: https://vancouver.ca/home-property-development/operating-permit.aspx
Clauß, M. (2006). Higher effectiveness of photoinactivation of bacterial spores, UV resistant vegetative bacteria and mold spores with 222 nm compared to 254 nm wavelength. Acta Hydrochim. Hydrobiol., 34(6): 525-532.
Cohn, P.D., Gleason, J.A., Rudowski, E, Tsai, S.M., Genese, C.A. and Faglianon, J.A. (2015). Community outbreak of legionellosis and an environmental investigation into a community water system. Epidemiol. Infect., 143(6): 1322–1331.
Collins, S., Stevenson, D., Bennett, A. and Walker, J. (2017). Occurrence of Legionella in UK household showers. Int. J. Hyg. Environ. Health, 220(2): 401-406.
Cope, J.R. and Ali, I.K. (2016). Primary amebic meningoencephalitis: What have we learned in the last 5 years? Curr. Infect. Dis. Rep., 18(10).
Correia, A.M., Gonçalves, J. and Gomes, J.P. (2016). Probable person-to-person transmission of legionnaires' disease. New Engl. J. Med., 374(5): 497-498.
Corti, M. and Palmero, D.J. (2008). Mycobacterium avium complex infection in HIV/AIDS patients. Expert Rev. Anti-Infect. Ther., 6(3): 351-363.
Covert, T.C., Rodgers, M.R., Reyes, A.L. and Stelma Jr., G.N. (1999). Occurrence of nontuberculous mycobacteria in environmental samples. Appl. Environ. Microbiol., 65(6): 2492-2496.
Craun, G.F., Brunkard, J.M., Yoder, J.S., Roberts, V.A., Carpenter, J., Wade, T., Calderon, R.L., Roberts, J.M., Beach, M.J. and Roy, S.L. (2010). Causes of outbreaks associated with drinking water in the United States from 1971 to 2006. Clin. Microbiol. Rev., 23(3): 507-528.
Croxen, M.A., Law, R.J., Scholz, R., Keeney, K.M., Wlodarska, M. and Finlay, B.B. (2013). Recent advances in understanding enteric pathogenic Escherichia coli. Clin. Microbiol. Rev., 26(4): 822-880.
Cunha, B.A., Burillo, A. and Bouza, E. (2016). Legionnaires' disease. Lancet, 387(10016): 376-385.
Cunha, C.B. and Cunha, B.A. (2017). Legionnaires’ disease since Philadelphia: Lessons learned and continued progress. Infect. Dis. Clin. North Am., 31(1): 1-5.
Daniels, T.L. and Gregory, D.W. (2015). Pseudomonas, Stenotrophomonas and Burkholderia. In Clinical infectious disease. Schlossberg, D. (ed.). Second edition. Cambridge University Press, Cambridge, pp. 966-974.
De Groote, M.A. (2004a). Disease resulting from contaminated equipment and invasive procedures. In Pathogenic mycobacteria in water—A guide to public health consequences, monitoring and management. Pedley, S. et al., (eds). World Health Organization, Geneva, Switzerland. pp: 131-142.
De Groote, M.A. (2004b). Pulmonary infection in non-HIV infected individuals. In Pathogenic mycobacteria in water—A guide to public health consequences, monitoring and management. Pedley, S. et al., (eds). World Health Organization, Geneva, Switzerland. pp: 115-130.
De Groote, M.A. and Johnson, P. (2004). Skin, bone and soft tissue infections. In Pathogenic mycobacteria in water—A guide to public health consequences, monitoring and management. Pedley, S. et al., (eds). World Health Organization, Geneva, Switzerland. pp: 104-114.
De Jonckheere, J.F. (2011). Origin and evolution of the worldwide distributed pathogenic amoeboflagellate Naegleria fowleri. Infect. Genet. Evol., 11(7): 1520-1528.
Degnan, A.J. (2006). Chapter 16. Pseudomonas. In AWWA manual of water supply practices—M48: Waterborne pathogens. 2nd edition. American Water Works Association, Denver, CO, pp. 131-134.
Dekker, J.P. and Frank, K.M. (2015). Salmonella, Shigella, and Yersinia. Clin. Lab. Med., 35(2): 225-246.
Devos, L., Boon, N. and Verstraete, W. (2005). Legionella pneumophila in the environment: The occurrence of a fastidious bacterium in oligotrophic conditions. Rev. Environ. Sci. Bio., 4 (1-2): 61-74.
Dias, V.C.F., Durand A-A., Constant P., Prévost M. and Bédard E. (2019) Identification of factors affecting bacterial abundance and community structures in a full-scale chlorinated drinking water distribution system. Water. 11(3): 627.
Dilger, T., Melzl, H. and Gessner, A. (2016). Legionella contamination in warm water systems in Germany—influence of maximal temperature and temperature during sampling. GWF Wasser Abwasser, 157(3): 262-271.
Dominguez, A., Alvarez, J., Sabria, M., Carmona, G., Torner, N., Oviedo, M., Cayla, J., Minguell, S., Barrabeig, I., Sala, M., Godoy, P. and Camps, N. (2009). Factors influencing the case-fatality rate of Legionnaires' disease. Int. J. Tuberc. Lung Dis., 13(3): 407-412.
Donohue, M.J., O'Connell, K., Vesper, S.J., Mistry, J.H., King, D., Kostich, M. and Pfaller, S. (2014). Widespread molecular detection of Legionella pneumophila serogroup 1 in cold water taps across the United States. Environ. Sci. Technol., 48(6): 3145-3152.
Donohue, M.J., Mistry, J.H., Donohue, J.M., Oconnell, K., King, D., Byran, J., Covert, T. and Pfaller, S. (2015). Increased frequency of nontuberculous mycobacteria detection at potable water taps within the United States. Environ. Sci. Technol., 49(10): 6127-6133.
Donohue, M.J. and Wymer, L. (2016). Increasing prevalence rate of nontuberculous mycobacteria infections in five states, 2008-2013. Annals of the American Thoracic Society, 13(12): 2143-2150.
Donohue, M.J., Vesper, S., Mistry, J. and Donohue, J.M. (2019a). Impact of chlorine and chloramine on the detection and quantification of Legionella pneumophila and Mycobacterium species. Appl. Environ. Microbiol., 85(24): e01942-19.
Donohue, M.J., King, D., Pfaller, S. and Mistry, J.H. (2019b). The sporadic nature of Legionella pneumophila, Legionella pneumophila Sg1 and Mycobacterium avium occurrence within residences and office buildings across 36 states in the United States. J. Appl. Microbiol., 126(5): 1568–1579.
ECDC (2017). European technical guidelines for the prevention, control and investigation, of infections caused by Legionella species. European Centre for Disease Prevention and Control (ECDC) and European Working Group for Legionella Infections (EWGLI). 125 pages. Accessed May 2021 from: https://www.ecdc.europa.eu/en/publications-data/european-technical-guidelines-prevention-control-and-investigation-infections.
ECDC. (2018a). European Centre for Disease Prevention and Control. Shigellosis. In ECDC. Annual epidemiological report for 2016. Stockholm: ECDC; 2018.
ECDC. (2018b). European Centre for Disease Prevention and Control. Yersiniosis. In ECDC. Annual epidemiological report for 2016. Stockholm: ECDC; 2018.
ECDC. (2019). European Centre for Disease Prevention and Control. Salmonellosis. In ECDC. Annual epidemiological report for 2016. Stockholm: ECDC; 2019.
Edberg, S.C., Browne, F.A. and Allen, M.J. (2007). Issues for microbial regulation: Aeromonas as a model. Crit. Rev. Microbiol., 33(1): 89-100.
Edelstein, P.H. and Roy, C.R. (2015). Legionnaires' disease and Pontiac fever. In Mandell, Douglas, and Bennett's principles and practice of infectious diseases. Bennett, J.E. et al. (eds.). Saunders. Philadelphia, USA. pp. 2633-2644.
Edelstein, P.H. (2007). Urine antigen tests positive for Pontiac fever: Implications for diagnosis and pathogenesis. Clin Infect Dis, 44(2): 229-231.
Egorov, A.I., Best, J.M., Frebis, C.P. and Karapondo, M.S. (2011). Occurrence of Aeromonas spp. in a random sample of drinking water distribution systems in the USA. J. Water. Health., 9(4): 785-798.
European Commission (2020). Directive (EU) 2020/2184 of the European Parliament and of the council of 16 December 2020 on the quality of water intended for human consumption (recast). Official Journal of the European Union. December 2020. Accessed August 2021 from: http://data.europa.eu/eli/dir/2020/2184/oj
Exner, M., Hartemann, P. and Lajoie, L (2007). Chapter 6. Health-care facilities. In Legionella and the prevention of legionellosis. Bartram, J. et al. (eds.). World Health Organization, Geneva, Switzerland. pp. 89-102.
Fagan-Garcia, K., Geary, J., Chang, H.-J., McAlpine, L., Walker, E., Colquhoun, A., Van Zanten, S.V., Girgis, S., Archie, B., Hanley, B., Corriveau, A., Morse, J., Munday, R., Goodman, K.J. (2019). Burden of disease from Helicobacter pylori infection in western Canadian Arctic communities. BMC Public Health, 19 (1), art. no. 730.
Falkinham, J.O. (2004). Environmental sources of Mycobacterium avium linked to routes of exposure. In Pathogenic mycobacteria in water A guide to public health consequences, monitoring and management. Pedley, S. et al (eds.). IWA Publishing. World Health Organization, Geneva, Switzerland. pp. 26-38.
Falkinham, J.O.,3rd (2015a). Common features of opportunistic premise plumbing pathogens. Int. J. Environ. Res. Public. Health., 12(5): 4533-4545.
Falkinham, J.O., III (2015b). Environmental sources of nontuberculous mycobacteria. Clinics in Chest Medicine, 36(1): 35-41.
Falkinham, J.O. (2015c). The Mycobacterium avium complex and slowly growing mycobacteria. In Molecular medical microbiology: Second edition. Elsevier Ltd, London, UK. pp. 1669-1678.
Falkinham, J.O.,3rd (2016a). Current epidemiologic trends of the nontuberculous mycobacteria (NTM). Curr Environ Health Rep, 3(2): 161-167.
Falkinham, J.O. (2016b). Nontuberculous mycobacteria: Community and nosocomial waterborne opportunistic pathogens. Clin. Microbiol. Newsl., 38(1): 1-7.
Falkinham III, J.O., Norton, C.D. and Lechevallier, M.W. (2001). Factors influencing numbers of Mycobacterium avium, Mycobacterium intracellulare, and other mycobacteria in drinking water distribution systems. Appl. Environ. Microbiol., 67(3): 1225-1231.
Falkinham, J.O. III, Hilborn, E.D., Arduino, M.J., Pruden, A. and Edwards, M.A. (2015a). Epidemiology and ecology of opportunistic premise plumbing pathogens: Legionella pneumophila, Mycobacterium avium, and Pseudomonas aeruginosa. Environ. Health Perspect., 123(8): 749-758.
Falkinham, J.O., Pruden, A. and Edwards, M. (2015b). Opportunistic premise plumbing pathogens: Increasingly important pathogens in drinking water. Pathogens, 4(2): 373-386.
Feazel, L.M., Baumgartner, L.K., Peterson, K.L., Frank, D.N., Harris, J.K. and Pace, N.R. (2009). Opportunistic pathogens enriched in showerhead biofilms. Proceedings of the National Academy of Sciences of the United States of America, 106(38): 16393-16398.
Fields, B.S., Haupt, T., Davis, J.P., Arduino, M.J., Miller, P.H. and Butler, J.C. (2001). Pontiac fever due to Legionella micdadei from a whirlpool spa: Possible role of bacterial endotoxin. J. Infect. Dis., 184(10): 1289-1292.
Fields, B.S., Benson, R.F. and Besser, R.E. (2002). Legionella and Legionnaires' disease: 25 years of investigation. Clin. Microbiol. Rev., 15(3): 506-526.
Fisman, D.N., Lim, S., Wellenius, G.A., Johnson, C., Britz, P., Gaskins, M., Maher, J., Mittleman, M.A., Spain, C.V., Haas, C.N. and Newbern, C. (2005). It's not the heat, it's the humidity: Wet weather increases legionellosis risk in the greater Philadelphia metropolitan area. J. Infect. Dis., 192(12): 2066-2073.
Fleury, M., Charron, D.F., Holt, J.D., Allen, O.B. and Maarouf, A.R. (2006). A time series analysis of the relationship of ambient temperature and common bacterial enteric infections in two Canadian provinces. Int. J. Biometeorol., 50 (6): 385-391.
Ford, T., Hermon-Taylor, J., Nichols, G., Cangelosi, G. and Bartram, J. (2004). Approaches to risk management in priority setting. In Pathogenic mycobacteria in Water—A guide to public health consequences, monitoring and management. Pedley, S. et al., (eds). World Health Organization, Geneva, Switzerland. pp. 169-178.
Fredriksson-Ahomaa, M. and Korkeala, H. (2003). Low occurrence of pathogenic Yersinia enterocolitica in clinical, food, and environmental samples: A methodological problem. Clin. Microbiol. Rev., 16(2): 220-229.
Fredriksson-Ahomaa, M. (2015). Enteropathogenic Yersinia spp. In Zoonoses-infections affecting humans and animals: Focus on public health aspects. Sing, A. (ed.). Springer. Dordrecht. pp. 213-234.
Fredriksson-Ahomaa, M. (2017). Yersinia enterocolitica. In Foodborne diseases: Third edition. Yersinia enterocolitica. Dodd, C. et al. (eds.). Academic Press. Cambridge, USA. pp. 223-233.
Friedman, M., Ashbolt, J., Hanson, A., Meteer, L. and Ureta, A. (2017). Chapter 3: Understanding and managing biofilm, coliform occurrence, and the microbial community. Manual of water supply practices M68– Water quality in distribution systems. American Water Works Association, Denver, CO., pp. 39-80.
Gagnon, G.A., Baribeau, H., Rutledge, S.O., Dumancic, R., Oehmen, A., Chauret, C. and Andrews, S. (2008). Disinfectant efficacy in distribution systems: a pilot-scale assessment. J. Water Supply Res. Technol. AQUA, 57(7): 507–518.
Gaia, V., Fry, N.K., Afshar, B., Lück, P.C., Meugnier, H., Etienne, J., Peduzzi, R. and Harrison, T.G. (2005). Consensus sequence-based scheme for epidemiological typing of clinical and environmental isolates of Legionella pneumophila. J. Clin. Microbiol., 43 (5):2047-2052.
Galanis, E., Mak, S., Otterstatter, M., Taylor, M., Zubel, M., Takaro, T.K., Kuo, M. and Michel, P. (2014). The association between campylobacteriosis, agriculture and drinking water: A case-case study in a region of British Columbia, Canada, 2005-2009. Epidemiol. Infect., 142 (10): 2075-2084.
Gargano, J.W., Adam, E.A., Collier, S.A., Fullerton, K.E., Feinman, S.J. and Beach, M.J. (2017). Mortality from selected diseases that can be transmitted by water—United States, 2003-2009. J. Water Health, 15(3): 438-450.
Garner, E., Brown, C.L., Schwake, D.O., Rhoads, W.J., Arango-Argoty, G., Zhang, L., Jospin, G., Coil, D.A., Eisen, J.A., Edwards, M.A. and Pruden, A. (2019). Comparison of whole-genome sequences of Legionella pneumophila in tap water and in clinical strains, Flint, Michigan, USA, 2016. Emerg. Infect. Dis., 25(11): 2013-2020.
Garrison, L.E., Kunz, J.M., Cooley, L.A., Moore, M.R., Lucas, C., Schrag, S., Sarisky, J. and Whitney, C.G. (2016). Vital signs: Deficiencies in environmental control identified in outbreaks of Legionnaires’ disease—North America, 2000–2014. Am. J. Transplant., 16 (10): 3049-3058.
Garvey, M., Thokala, N. and Rowan, N. (2014). A comparative study on the pulsed UV and the low-pressure UV inactivation of a range of microbial species in water. Water Environ. Res., 86(12): 2317-2324.
Gavriel, A.A., Landre, J.P.B. and Lamb, A.J. (1998). Incidence of mesophilic Aeromonas within a public drinking water supply in north-east Scotland. J. Appl. Microbiol., 84(3): 383-392.
Gebert, M.J., Delgado-Baquerizo, M., Oliverio, A.M., Webster, T.M., Nichols, L.M., Honda, J.R., Chan, E.D., Adjemian, J., Dunn, R.R. and Fierer, N. (2018). Ecological analyses of mycobacteria in showerhead biofilms and their relevance to human health. mBio, 9(5).
George, K.L., Parker, B.C., Gruft, H. and Falkinham III, J.O. (1980). Epidemiology of infection by nontuberculous mycobacteria. II. Growth and survival in natural waters. Am. Rev. Respir. Dis., 122 (1): 89-94.
Gerba, C.P., Nwachuku, N. and Riley, K.R. (2003). Disinfection resistance of waterborne pathogens on the United States Environmental Protection Agency's Contaminant candidate list (CCL). J. Water Supply Res. T., 52(2): 81-94.
Ghenghesh, K.S., Ahmed, S.F., El-Khalek, R.A., Al-Gendy, A. and Klena, J. (2008). Aeromonas-associated infections in developing countries. J. Infect. Dev. Countr., 2(2): 81-98.
Gillespie, S., Lipphaus, P., Green, J., Parsons, S., Weir, P., Juskowiak, K., Jefferson, B., Jarvis, P. and Nocker, A. (2014). Assessing microbiological water quality in drinking water distribution systems with disinfectant residual using flow cytometry. Water Res., 65: 224–234.
Gilmour, M.W., Bernard, K., Tracz, D.M., Olson, A.B., Corbett, C.R., Burdz, T., Ng, B., Wiebe, D., Broukhanski, G., Boleszczuk, P., Tang, P., Jamieson, F., Van Domselaar, G., Plummer, F.A. and Berry, J.D. (2007). Molecular typing of a Legionella pneumophila outbreak in Ontario, Canada. J. Med. Microbiol., 56(3): 336-341.
Goldberg, D., Collier, P., Fallon, R., Mckay, T., Markwick, T., Wrench, J., Emslie, J., Forbes, G., Macpherson, A. and Reid, D. (1989). Lochgoilhead fever: Outbreak of non-pneumonic legionellosis due to Legionella micdadei. Lancet, 333(8633): 316-318.
Gora, S.L., Anaviapik Soucie, T., McCormick, N.E., Ontiveros, C.C., L’Hérault, V., Gavin, M., Trueman, B.F., Campbell, J. Stoddard, A.K. and Gagnon, G.A. (2020). Microbiological water quality in a decentralized Arctic drinking water system. Environ. Sci.: Water Res. Technol., 6(7): 1855–1868.
Gorski, L., Rivadeneira, P. and Cooley, M.B. (2019). New strategies for the enumeration of enteric pathogens in water. Env. Microbiol. Rep., 11 (6): 765-776.
Goudot, S., Herbelin, P., Mathieu, L., Soreau, S., Banas, S. and Jorand, F.P.A. (2014). Biocidal efficacy of monochloramine against planktonic and biofilm-associated Naegleria fowleri cells. J. Appl. Microbiol., 116(4): 1055-1065.
Government Inquiry into Havelock North Drinking Water. (2017). Havelock North drinking water inquiry: Stage 2. May 2017, Auckland, New Zealand. ISBN: 978-0-473-39743-2. Available at https://www.dia.govt.nz/stage-1-of-the-water-inquiry.
Government of Quebec. (2020). Régie du Bâtiment Québec. Quebec, Canada. Accessed February, 2020 from: https://www.rbq.gouv.qc.ca/domaines-dintervention/batiment/interpretation-directives-techniques-et-administratives/chapitre-batiment-du-code-de-securite/tours-de-refroidissement-a-leau-obligations-du-proprietaire.html
Graham, D.Y., Opekun, A.R., Osato, M.S., El-Zimaity, H.M.T., Lee, C.K., Yamaoka, Y., Qureshi, W.A., Cadoz, M. and Monath, T.P. (2004). Challenge model for Helicobacter pylori infection in human volunteers. Gut, 53(9): 1235-1243.
Graziani, C., Losasso, C., Luzzi, I., Ricci, A., Scavia, G. and Pasquali, P. (2017). Salmonella. In Foodborne diseases: Third edition. Dodd, C. et al. (eds.). Academic Press. Cambridge, USA. pp. 133-169.
Greenberg, D., Chiou, C.C., Famigilleti, R., Lee, T.C. and Yu, V.L. (2006). Problem pathogens: Paediatric legionellosis-implications for improved diagnosis. Lancet Infect. Dis., 6(8): 529-535.
Griffin, I. Schmitz, A., Oliver, C., Pritchard, S., Zhang, G., Rico, E., Davenport, E., Llau, A., Moore, E., Fernandez, D., Mejia-Echeverry, A., Suarez, J., Noya-Chaveco, P., Elmir, S., Jean, R., Pettengill, J.B., Hollinger, K.A., Chou, K., Williams-Hill, D., .… and L. Rivera, L. (2019). Outbreak of Tattoo-associated nontuberculous mycobacterial skin infections. Clin Infect Dis. 69(6):949-955.
Griffith, D.E., Aksamit, T., Brown-Elliott, B.A., Catanzaro, A., Daley, C., Gordin, F., Holland, S.M., Horsburgh, R., Huitt, G., Iademarco, M.F., Iseman, M., Olivier, K., Ruoss, S., Von Reyn, C.F., Wallace Jr., R.J. and Winthrop, K. (2007). An official ATS/IDSA statement: Diagnosis, treatment, and prevention of nontuberculous mycobacterial diseases. Am. J. Resp. Crit. Care, 175 (4):367-416.
Grimont, P.A.D. and Weill, F-X. (2007). Antigenic formulae of the Salmonella serovars. 9th edition. WHO Collaborating Centre for Reference and Research on Salmonella. Institut Pasteur, Paris, France. Accessed January 2020 from: https://www.pasteur.fr/sites/default/files/veng_0.pdf
Guimaraes, A.J., Gomes, K.X., Cortines, J.R., Peralta, J.M. and Peralta, R.H.S. (2016). Acanthamoeba spp. as a universal host for pathogenic microorganisms: One bridge from environment to host virulence. Microbiol. Res., 193: 30-38.
Hallenbeck, W.H. and Brenniman, G.R. (1989). Risk of fatal amebic meningoencephalitis from waterborne Naegleria fowleri. Environ. Manage., 13(2): 227-232.
Halstrom, S., Price, P. and Thomson, R. (2015). Review: Environmental mycobacteria as a cause of human infection. Int. J. Mycobacteriology, 4(2): 81-91.
Hamilton, K.A., Weir, M.H. and Haas, C.N. (2017). Dose response models and a quantitative microbial risk assessment framework for the Mycobacterium avium complex that account for recent developments in molecular biology, taxonomy, and epidemiology. Water Res., 109: 310-326.
Handfield, M., Simard, P., Couillard, M. and Letarte, R. (1996). Aeromonas hydrophila isolated from food and drinking water: Hemagglutination, hemolysis, and cytotoxicity for a human intestinal cell line (HT-29). Appl. Environ. Microbiol., 62(9): 3459-3461.
Harris, N.B. and Barletta, R.G. (2001). Mycobacterium avium subsp. paratuberculosis in Veterinary Medicine. Clin. Microbiol. Rev., 14 (3):489-512.
Havelaar, A.H., Schets, F.M., van Silfhout, A., Jansen, W.H., Wieten, G. and van der Kooij, D. (1992). Typing of Aeromonas strains from patients with diarrhoea and from drinking water. J. Appl. Bacteriol., 72(5): 435-444.
Hayes, S.L., White, K.M. and Rodgers, M.R. (2006). Assessment of the effectiveness of low-pressure UV light for inactivation of Helicobacter pylori. Appl. Environ. Microbiol., 72(5): 3763-3765.
Hayes, S.L., Sivaganesan, M., White, K.M. and Pfaller, S.L. (2008). Assessing the effectiveness of low-pressure ultraviolet light for inactivating Mycobacterium avium complex (MAC) micro-organisms. Lett. Appl. Microbiol., 47(5): 386-392.
Health Canada. (2019a). Be Well Aware—Information for private well owners. Healthy Environments and Consumer Safety Branch, Health Canada. Ottawa, Ontario. Available at https://www.canada.ca/en/health-canada/services/publications/healthy-living/water-talk-information-private-well-owners.html
Health Canada. (2019b). Guidelines for Canadian Drinking Water Quality: Guideline Technical Document—Enteric protozoa: Giardia and Cryptosporidium. Water, and Air Quality Bureau, Healthy Environments and Consumer Safety Branch, Health Canada, Ottawa, Ontario. Available at https://www.canada.ca/en/health-canada/services/environmental-workplace-health/reports-publications/water-quality/enteric-protozoa-giardia-cryptosporidium.html
Health Canada. (2019c). Guidelines for Canadian Drinking Water Quality: Guideline Technical Document—Enteric Viruses. Water, Air and Climate Change Bureau, Healthy Environments and Consumer Safety Branch, Health Canada, Ottawa, Ontario. Available at: https://www.canada.ca/en/health-canada/services/publications/healthy-living/guidelines-canadian-drinking-water-quality-guideline-technical-document-enteric-viruses.html
Health Canada (2020a). Guidance on natural organic matter in drinking water. Water Quality and Health Bureau, Healthy Environments and Consumer Safety Branch, Health Canada, Ottawa, Ontario. Available at: www.canada.ca/en/health-canada/services/publications/healthy-living/guidance-natural-organic-matter-drinking-water.html
Health Canada (2020b). Guidelines for Canadian Drinking Water Quality: Guideline Technical Document — Chloramines. Water and Air Quality Bureau, Healthy Environments and Consumer Safety Branch, Health Canada, Ottawa, Ontario. Available at https://www.canada.ca/en/health-canada/services/publications/healthy-living/guidelines-canadian-drinking-water-quality-guideline-technical-document-chloramines.html
Health Canada. (2020c). Guidelines for Canadian Drinking Water Quality: Guideline Technical Document—Escherichia coli in Drinking Water. Water and Air Quality Bureau, Healthy Environments and Consumer Safety Branch, Health Canada, Ottawa, Ontario. Available at https://www.canada.ca/en/health-canada/services/publications/healthy-living/guidelines-canadian-drinking-water-quality-guideline-technical-document-escherichia-coli.html
Health Canada (2021). Guidance on the temperature aspects of drinking water. Water Quality and Health Bureau, Healthy Environments and Consumer Safety Branch, Health Canada, Ottawa, Ontario.
Health Canada (2022). Guidance on monitoring the biological stability of drinking water in distribution systems. Water Quality and Health Bureau, Healthy Environments and Consumer Safety Branch, Health Canada, Ottawa, Ontario.
Henkle, E., Hedberg, K., Schafer, S.D. and Winthrop, K.L. (2017). Surveillance of extrapulmonary nontuberculous mycobacteria infections, Oregon, USA, 2007–2012. Emerg. Infect. Dis., 23(10): 1627-1630.
Hermon-Taylor., J. and El-Zaatari, F.A.K. (2004). The Mycobacterium avium subspecies paratuberculosis problem and its relation to the causation of Crohn’s disease. In Pathogenic mycobacteria in Water—A guide to public health consequences, monitoring and management. Pedley, S. et al., (eds). World Health Organization, Geneva, Switzerland. pp: 74-94.
Hijnen, W.A.M. and Medema, G.J. (2010). Elimination of micro-organisms by drinking water treatment processes. KWR Watercycle Research Institute. IWA Publishing, London, United Kingdom. Pp. 1-102.
Hijnen, W.A.M., Beerendonk, E.F. and Medema, G.J. (2011). Inactivation credit of UV radiation for viruses, bacteria and protozoan (oo)cysts in water: A review.). In Quantitative methods to assess capacity of water treatment to eliminate microorganisms. Hijnen, W.A.M ed.; KWR Watercycle Research Institute. IWA Publishing., London, United Kingdom.
Hilborn, E.D., Covert, T.C., Yakrus, M.A., Harris, S.I., Donnelly, S.F., Rice, E.W., Toney, S., Bailey, S.A. and Stelma Jr., G.N. (2006). Persistence of nontuberculous mycobacteria in a drinking water system after addition of filtration treatment. Appl. Environ. Microbiol. , 72(9): 5864-5869.
Hlavsa, M.C., Cikesh, B.L., Roberts, V.A., Kahler, A.M., Vigar, M., Hilborn, E.D., Wade, T.J., Roellig, D.M., Murphy, J.L., Xiao, L., Yates, K.M., Kunz, J.M., Arduino, M.J., Reddy, S.C., Fullerton, K.E., Cooley, L.A., Beach, M.J., Hill, V.R. and Yoder, J.S. (2018). Outbreaks associated with treated recreational water—United States, 2000–2014. Am. J. Transplant., 18(7): 1815-1819.
Hoefsloot, W. et al. (2013). The geographic diversity of nontuberculous mycobacteria isolated from pulmonary samples: An NTM-NET collaborative study. European Respiratory Journal, 42(6): 1604-1613.
Holinger, E.P., Ross, K.A., Robertson, C.E., Stevens, M.J., Harris, J.K. and Pace, N.R. (2014). Molecular analysis of point-of-use municipal drinking water microbiology. Water Res., 49: 225-235.
Holme, R. (2003). Drinking water contamination in Walkerton, Ontario: Positive resolutions from a tragic event. Wat. Sci. Technol. 47 (3):1-6.
Hooi, J.K.Y., Lai, W.Y., Ng, W.K., Suen, M.M.Y., Underwood, F.E., Tanyingoh, D., Malfertheiner, P., Graham, D.Y., Wong, V.W.S., Wu, J.C.Y., Chan, F.K.L., Sung, J.J.Y., Kaplan, G.G. and Ng, S.C. (2017). Global Prevalence of Helicobacter pylori Infection: Systematic Review and Meta-Analysis. Gastroenterology, 153 (2): 420-429.
Hornei, B., Ewig, S., Exner, M., Tartakovsky, I., Lajoie, L., Dangendorf, F., Surman-Lee, S. and Fields, B. (2007). Chapter 1: Legionellosis. In Legionella and the prevention of legionellosis. Bartram, J. et. al. (eds.). World Health Organization, Geneva, Switzerland. pp. 1-27.
Howden, B.P., Stuart, R.L., Tallis, G., Bailey, M. and Johnson, P.D.R. (2003). Treatment and outcome of 104 hospitalized patients with legionnaires’ disease. Internal Medicine Journal, 33(11): 484-488.
Hrudey, S. E. and Hrudey, E. (2004). Safe drinking water: Lessons from recent outbreaks in affluent nations. Safe drinking water: Lessons from recent outbreaks in affluent nations. IWA Publishing, London.
HSE. (2013a). Legionnaires’ Disease: Technical Guidance. HSG274 Part 1: The Control of Legionella Bacteria in Evaporative Cooling Systems. Health and Safety Executive, Government of the United Kingdom. London, UK. Pp. 1-57. Accessed December 2018 from: http://www.hse.gov.uk/pubns/books/hsg274.htm
HSE. (2013b). Legionnaires' Disease. The Control of Legionella Bacteria in Water Systems—Approved Code of Practice and Guidance. L8 (Fourth Edition). Health and Safety Executive, Government of the United Kingdom. London, UK. Pp. 1-28. Accessed November 2018 from: http://www.hse.gov.uk/pubns/books/l8.htm
HSE. (2014). Legionnaires’ Disease: Technical Guidance. HSG274 Part 2: The Control of Legionella Bacteria in Hot and Cold Water Systems. Health and Safety Executive, Government of the United Kingdom. London, UK. Pp. 1-65. Accessed December 2018 from: http://www.hse.gov.uk/pubns/books/hsg274.htm
HSE. (2019). Legionella and Legionnaires' Disease – Frequently asked questions. Health and Safety Executive, Government of the United Kingdom. London, UK. Accessed November 2018 from: http://www.hse.gov.uk/legionnaires/faqs.htm#Testing-monitoring
Hsu, M.-S., Wu, M.-Y., Huang, Y.-T. and Liao, C.-H. (2016). Efficacy of chlorine dioxide disinfection to non-fermentative gram-negative bacilli and non-tuberculous mycobacteria in a hospital water system. Journal of Hospital Infection, 93(1): 22-28.
Huang, H., Brooks, B.W., Lowman, R. and Carrillo, C.D. (2015). Campylobacter species in animal, food, and environmental sources, and relevant testing programs in Canada. Can. J. Microbiol., 61(10): 701-721.
Hull, N.M., Holinger, E.P., Ross, K.A., Robertson, C.E., Harris, J.K, Stevens, M.J. and Pace, N.R. (2017). Longitudinal and Source-to-Tap New Orleans, LA, U.S.A. Drinking Water Microbiology. Environ. Sci. Technol., 51(8): 4220–4229.
IARC Helicobacter pylori Working Group. (2014). Helicobacter pylori eradication as a strategy for preventing gastric cancer. International Agency for Research on Cancer, IARC Working Group Reports, No. 8, Lyon, France.
Ibranosyan, M., Beraud, L., Lemaire, H., Ranc, A-G., Ginevra, C., Jarraud, S. and Descours, G. (2019). The clinical presentation of Legionella arthritis reveals the mode of infection and the bacterial species: Case report and literature review. BMC Infect. Dis., 19(1) art. no. 864.
Ishii, S. and Sadowsky, M.J. (2008). Escherichia coli in the environment: Implications for water quality and human health. Microbes Environ., 23(2): 101-108.
ISO. (2019). ISO ICS 07.100.20 – Microbiology of Water. International Organization for Standardization. Geneva, Switzerland. Accessed from: www.iso.org/Ics/07.100.20/X/.
Jacangelo, J.G., Patania, N.L., Trussell, R.R., Haas, C.N. and Gerba, C. (2002). Inactivation of waterborne emerging pathogens by selected disinfectants. AWWA Research Foundation and American Water Works Association, Denver, CO.
Jamil, A., Farooq, S. and Hashmi, I. (2017). Ozone disinfection efficiency for indicator microorganisms at different pH values and temperatures. Ozone Sci. Eng., 39(6): 407-416.
Janda, J.M. and Abbott, S.L. (2010). The genus Aeromonas: Taxonomy, pathogenicity, and infection. Clinical Microbiology Reviews, 23(1): 35-73.
Johnson, C.H., Rice, E.W. and Reasoner, D.J. (1997). Inactivation of Helicobacter pylori by chlorination. Appl. Environ. Microbiol., 63(12): 4969-4970.
Jones, T.F., Benson, R.F., Brown, E.W., Rowland, J.R., Crosier, S.C. and Schaffner, W. (2003). Epidemiologic investigation of a restaurant-associated outbreak of pontiac fever. Clin Infect Dis, 37(10): 1292-1297.
Juárez, M.M., Tártara, L.I., Cid, A.G., Real, J.P., Bermúdez, J.M., Rajal, V.B. and Palma, S.D. (2018). Acanthamoeba in the eye, can the parasite hide even more? latest developments on the disease. Contact Lens and Anterior Eye, 41(3): 245-251.
Kaakoush, N.O., Castaño-Rodríguez, N., Mitchell, H.M. and Man, S.M. (2015). Global epidemiology of Campylobacter infection. Clinical Microbiology Reviews, 28(3): 687-720.
Kanamori, H., Weber, D.J. and Rutala, W.A. (2016). Healthcare outbreaks associated with a water reservoir and infection prevention strategies. Clin. Infect. Dis., 62(11): 1423-1435.
Katz, M.J., Parrish, N.M., Belani, A. and Shah, M. (2015). Recurrent Aeromonas bacteremia due to contaminated well water. Open Forum Infectious Diseases, 2(4).
Kaur, S. (2014). Chapter 9: Pathogenic mycobacteria and water. In Water and health. Singh, P.P. and Sharma, V. (eds.) Springer. India. pp. 137-153.
Keithlin, J., Sargeant, J., Thomas, M.K. and Fazil, A. (2014a). Chronic sequelae of E. coli O157: Systematic review and meta-analysis of the proportion of E. coli O157 cases that develop chronic sequelae. Foodborne Pathog. Dis., 11 (2): 79-95.
Keithlin, J., Sargeant, J., Thomas, M.K. and Fazil, A. (2014b). Systematic review and meta-analysis of the proportion of Campylobacter cases that develop chronic sequelae. BMC Public Health, 14 (1), art. no. 1203: 1-19.
Keithlin, J., Sargeant, J.M., Thomas, M.K. and Fazil, A. (2015). Systematic review and meta-analysis of the proportion of non-typhoidal Salmonella cases that develop chronic sequelae. Epidemiol. Infect., 143: 1333–1351.
Kennedy, D. and Wilkinson, M.G. (2017). Application of flow cytometry to the detection of pathogenic bacteria. Curr. Issues Mol. Biol., 23: 21-38.
Khajanchi, B.K., Fadl, A.A., Borchardt, M.A., Berg, R.L., Horneman, A.J., Stemper, M.E., Joseph, S.W., Moyer, N.P., Sha, J. and Chopra, A.K. (2010). Distribution of virulence factors and molecular fingerprinting of Aeromonas species isolates from water and clinical samples: Suggestive evidence of water-to-human transmission. Appl. Environ. Microbiol., 76(7): 2313-2325.
Kilvington, S., Gray, T., Dart, J., Morlet, N., Beeching, J.R., Frazer, D.G. and Matheson, M. (2004). Acanthamoeba keratitis: The role of domestic tap water contamination in the United Kingdom. Invest. Ophth. Vis. Sci., 45(1): 165-169.
King, D.N., Donohue, M.J., Vesper, S.J., Villegas, E.N., Ware, M.W., Vogel, M.E., Furlong, E.F., Kolpin, D.W., Glassmeyer, S.T. and Pfaller, S. (2016). Microbial pathogens in source and treated waters from drinking water treatment plants in the United States and implications for human health. Sci. Total Environ., 562: 987–995.
Knøchel, S. (1991). Chlorine resistance of motile Aeromonas spp. Water Sci. Technol., 24(2): 327-330.
Knox, N.C., Weedmark, K.A., Conly, J., Ensminger, A.W., Hosein, F.S. and Drews, S.J. (2017). Unusual Legionnaires' outbreak in cool, dry western Canada: An investigation using genomic epidemiology. Epidemiol. Infect., 145(2): 254-265.
Köhsler, M., Mrva, M. and Walochnik, J. (2016). Acanthamoeba. In Molecular parasitology: Protozoan parasites and their molecules. Walochnik, J. and Duchêne, M. (eds.). Springer. Vienna. pp. 285-324.
Kothary, M.H. and Babu, U.S. (2001). Infective dose of foodborne pathogens in volunteers: A review. J. Food Safety, 21(1): 49-73.
Kuchta J.M., States S.J., McNamara A.M., Wadowsky R.M., and Yee R.B. (1983). Susceptibility of Legionella pneumophila to chlorine in tap water. Appl. Environ. Microbiol., 46:1134–1139
Kühn, I., Allestam, G., Huys, G., Janssen, P., Kersters, K., Krovacek, K. and Stenström, T-A. (1997). Diversity, persistence, and virulence of Aeromonas strains isolated from drinking water distribution systems in Sweden. Appl. Environ. Microbiol., 63(7): 2708-2715.
Kvalsvig, A., Baker, M.G., Sears, A. and French, N. (2013). Bacteria: Campylobacter. In Encyclopedia of food safety. Motarjemi, Y., Moy, G. and Todd, E. (eds.). Academic Press. Cambridge, USA. pp. 369-380.
Lal, A., Hales, S., French, N., and Baker, M.G. (2012). Seasonality in human zoonotic enteric diseases: A systematic review. PLoS ONE, 7 (4), art. no. e31883.
Lande, L., Alexander, D.C., Wallace, R.J., Jr., Kwait, R., Iakhiaeva, E., Williams, M., Cameron, A.D.S., Olshefsky, S., Devon, R., Vasireddy, R., Peterson, D.D. and Falkinham, J.O., III (2019). Mycobacterium avium in community and household water, suburban Philadelphia, Pennsylvania, USA, 2010-2012. Emerg. Infect. Dis., 25(3): 473-481.
Langenberg, W., Rauws, E.A.J., Oudbier, J.H. and Tytgat, G.N.J. (1990). Patient-to-patient transmission of Campylobacter pylori infection by fiber optic gastroduodenoscopy and biopsy. J. Infect. Dis., 161(3): 507-511.
Lau, H.Y. and Ashbolt, N.J. (2009). The role of biofilms and protozoa in Legionella pathogenesis: Implications for drinking water. J. Appl. Microbiol., 107 (2): 368-378.
Lavenir, R., Sanroma, M., Gibert, S., Crouzet, O., Laurent, F., Kravtsoff, J., Mazoyer, M-A. and Cournoyer, B. (2008). Spatio-temporal analysis of infra-specific genetic variations among a Pseudomonas aeruginosa water network hospital population: Invasion and selection of clonal complexes. J. Appl. Microbiol., 105(5):1491-1501.
Le Dantec, C., Duguet, J-P., Montiel, A., Dumoutier, N., Dubrou, S. and Vincent, V. (2002). Occurrence of mycobacteria in water treatment lines and in water distribution systems. Appl. Environ. Microbiol., 68(11): 5318-5325.
LeChevallier, M.W. (2004). Control, treatment and disinfection of Mycobacterium avium complex in drinking water. In Pathogenic mycobacteria in water—A guide to public health consequences, monitoring and management. Pedley, S. et al., (eds). World Health Organization, Geneva, Switzerland. pp: 143-168.
LeChevallier, M.W. (2019a). Monitoring distribution systems for Legionella pneumophila using Legiolert. AWWA Wat Sci., 1(1): e1122.
LeChevallier, M.W. (2019b). Occurrence of culturable Legionella pneumophila in drinking water distribution systems. AWWA Wat Sci., 1(3): e1139.
LeChevallier, M.W. (2020). Managing Legionella pneumophila in water systems. J. Am. Water Works Assoc., 112(2): 10–23.
LeChevallier, M.W. and Au, K.K. (2004). Water treatment and pathogen control: Process efficiency in achieving safe drinking water. IWA Publishing, London, UK, on behalf of the World Health Organization, Geneva, Switzerland.
LeChevallier, M.W., Evans, T.M., Seidler, R.J., Daily, O.P., Merrell, B.R., Rollins, D.M. and Joseph, S.W. (1982). Aeromonas sobria in chlorinated drinking water supplies. Microb. Ecol., 8(4): 325-333.
LeChevallier, M.W., Norton, C.D., Falkinham, III, J.O., Williams, M.D., Taylor, R.H. and Cowan, H.E. (2001). Occurrence and control of Mycobacterium avium complex. AWWA Research Foundation and American Water Works Association., Denver. CO.
LeChevallier, M.W., Schneider, O.D., Weinrich, L.A., Jjemba, P.K., Evans, P.J., Hooper, J.L. and Chappell, R.W. (2015a). An operational definition of biostability in drinking water. Water Research Foundation, Denver, Colorado.
LeChevallier, M.W., Schneider, O.D., Weinrich, L.A., Jjemba, P.K., Evans, P.J., Hooper, J.L. and Chappell, R.W. (2015b). Guidance manual for control of biostability in drinking water. Water Research Foundation, Denver, Colorado.
Leclerc, H. (2003). Relationships between common water bacteria and pathogens in drinking-water. In Heterotrophic plate counts and drinking-water safety. The significance of HPCs for water quality and human health. Bartram, J., Cotruvo, J., Exner, M., Fricker, C. and Glasmacher, A. (eds.). IWA Publishing, London, UK. pp. 80–118.
Lee, G., Pan, W., Peñataro Yori, P., Paredes Olortegui, M., Tilley, D., Gregory, M., Oberhelman, R., Burga, R., Chavez, C.B. and Kosek, M. (2013). Symptomatic and asymptomatic Campylobacter infections associated with reduced growth in Peruvian children. PLoS Neglect. Trop. D., 7 (1), art. no. e2036.
Leoni, E., Catalani, F., Marini, S. and Dallolio, L. (2018). Legionellosis associated with recreational waters: A systematic review of cases and outbreaks in swimming pools, spa pools, and similar environments. Int. J. Env. Res. Pub. He., 15 (8), art. no. 1612.
Lévesque, S., Plante, P-L., Mendis, N., Cantin, P., Marchand, G., Charest, H., Raymond, F., Huot, C., Goupil-Sormany, I., Desbiens, F., Faucher, S.P., Corbeil, J. and Tremblay, C. (2014). Genomic characterization of a large outbreak of Legionella pneumophila serogroup 1 strains in Quebec City, 2012. PLoS ONE, 9(8).
Levy, K., Woster, A.P., Goldstein, R.S. and Carlton, E.J. (2016). Untangling the impacts of climate change on waterborne diseases: A systematic review of relationships between diarrheal diseases and temperature, rainfall, flooding, and drought. Environ. Sci. Technol., 50(10): 4905-4922.
Li, T., Abebe, L.S., Cronk, R. and Bartram, J. (2017). A systematic review of waterborne infections from nontuberculous mycobacteria in health care facility water systems. Int. J. Hyg. Envir. Heal., 220(3): 611-620.
Li, J., Zhu, Y., Wu, X. and Hoffmann, M.R. (2020). Rapid detection methods for bacterial pathogens in ambient waters at the point of sample collection: A brief review. Clin. Infect. Dis., 71:S84-S90.
Liu, D. (2014). Aeromonas. In Molecular medical microbiology: Second edition. Tang, Y-W. et al., eds. Elsevier Ltd. London, UK. pp. 1099-1110.
Llewellyn, A.C., Lucas, C.E., Roberts, S.E., Brown, E.W., Nayak, B.S., Raphael, B.H. and Winchell, J.M. (2017). Distribution of Legionella and bacterial community composition among regionally diverse U.S. cooling towers. PLoS ONE, 12 (12).
Loret, J-F. and Greub, G. (2010). Free-living amoebae: Biological by-passes in water treatment. Int. J. Hyg. Envir. Heal., 213(3): 167-175.
Loret, J-F., Jousset, M., Robert, S., Anselme, C., Saucedo, G., Ribas, F., Martinez, L. and Catalan, V. (2008). Elimination of free-living amoebae by drinking water treatment processes. Journal Europeen D'Hydrologie, 39(1): 37-50.
Louisiana Department of Health and Hospitals (2013). Declaration of emergency: Minimum disinfectant residual in public water systems. Office of Public Health, State of Louisiana. Accessed December 2021 from: https://ldh.la.gov/assets/oph/EmergencyRule/EmergencyRule-MinimumDisinfectantResidualLevels.pdf
LPSN. (2019). List of prokaryote names with standing in nomenclature. Retrieved June 2019, from www.bacterio.net.
Lu, J., Struewing, I., Yelton, S. and Ashbolt, N. (2015). Molecular survey of occurrence and quantity of Legionella spp., Mycobacterium spp., Pseudomonas aeruginosa and amoeba hosts in municipal drinking water storage tank sediments. J. Appl. Microbiol., 119(1): 278-288.
Lu, J., Struewing, I., Vereen, E., Kirby, A.E., Levy, K., Moe, C. and Ashbolt, N. (2016). Molecular detection of Legionella spp. and their associations with Mycobacterium spp., Pseudomonas aeruginosa and amoeba hosts in a drinking water distribution system. J. Appl. Microbiol., 120(2): 509-521.
Lund, V. (1996). Evaluation of E. coli as an indicator for the presence of Campylobacter jejuni and Yersinia enterocolitica in chlorinated and untreated oligotrophic lake water. Water Res., 30(6): 1528-1534.
Lüttichau, H.R., Vinther, C., Uldum, S.A., Møller, J., Faber, M. and Jensen, J.S. (1998). An outbreak of Pontiac fever among children following use of a whirlpool. Clin. Infect. Dis., 26(6): 1374-1378.
MacIntyre, C.R., Dyda, A., Bui, C.M. and Chughtai, A.A. (2018). Rolling epidemic of Legionnaires' disease outbreaks in small geographic areas. Emerg. Microbes Infect., 7(1): 36.
Magnet, A., Galván, A.L., Fenoy, S., Izquierdo, F., Rueda, C., Fernandez Vadillo, C., Pérez-Irezábal, J., Bandyopadhyay, K., Visvesvara, G.S., Da Silva, A.J. and Del Aguila, C. (2012). Molecular characterization of Acanthamoeba isolated in water treatment plants and comparison with clinical isolates. Parasitol. Res., 111(1): 383-392.
Mao, G., Song, Y., Bartlam, M. and Wang, Y. (2018). Long-term effects of residual chlorine on Pseudomonas aeruginosa in simulated drinking water fed with low AOC medium. Front. Microbiol., 9(MAY).
Marciano-Cabral, F. and Cabral, G.A. (2007). The immune response to Naegleria fowleri amebae and pathogenesis of infection. FEMS Immunol. Med. Mic., 51(2): 243-259.
Marras, T.K., Mendelson, D., Marchand-Austin, A., May, K. and Jamieson, F.B. (2013). Pulmonary nontuberculous mycobacterial disease, Ontario, Canada, 1998-2010. Emerg. Infect. Dis., 19(11): 1889-1891.
Marrie, T.J., De Carolis, E., Yu, V.L. and Stout, J. (2003). Legionnaires' disease - results of a multicentre Canadian study. Can. J. Infect. Dis., 14(3): 154-158.
Massa, S., Armuzzi, R., Tosques, M., Canganella, F. and Trovatelli, L.D. (1999). Note: Susceptibility to chlorine of Aeromonas hydrophila strains. J. Appl. Microbiol. 86 (1):169-173.
Masters, S., Clancy, J., Villegas, S., LeChevallier, M. and Bukhari, Z (2019). Customer Messaging on Plumbing System Issues. Project #4664. Water Research Foundation Denver, Colo.
Mathys, W., Stanke, J., Harmuth, M. and Junge-Mathys, E. (2008). Occurrence of Legionella in hot water systems of single-family residences in suburbs of two German cities with special reference to solar and district heating. Int. J. Hyg. Environ. Heal., 211 (1-2): 179-185.
Matysiak-Budnik, T., Briet, F., Heyman, M. and Mégraud, F. (1995). Laboratory-acquired Helicobacter pylori infection. Lancet, 346(8988): 1489-1490.
McDermott, P.F., Zhao, S. and Tate, H. (2018). Antimicrobial Resistance in Nontyphoidal Salmonella. Microbiology spectrum, American Society for Microbiology. 6(4):1-26.
McDonough, E.A., Metzgar, D., Hansen, C.J., Myers, C.A. and Russell, K.L. (2007). A cluster of Legionella-associated pneumonia cases in a population of military recruits. J. Clin. Microbiol., 45(6): 2075-2077.
MDHHS. (2016). Legionellosis outbreak-Genesee County, June, 2014 – March, 2015. Full analysis. Michigan Department of Health and Human Services, MI. Accessed November 2018 from www.michigan.gov.
Medema, G.J., Wondergem, E., Van Dijk-Looyaard, A.M. and Havelaar, A.H. (1991). Effectivity of chlorine dioxide to control Aeromonas in drinking water distribution systems. Water Sci. Technol., 24(2): 325-326.Mégraud, F. and Lehours, P. (2007). Helicobacter pylori detection and antimicrobial susceptibility testing (2007) Clin. Microbiol. Rev., 20 (2): 280-322.
Mercante, J.W. and Winchell, J.M. (2015). Current and emerging Legionella diagnostics for laboratory and outbreak investigations. Clin. Microbiol. Rev., 28(1): 95-133.
Mir, R.A. and Kudva, I.T. (2019). Antibiotic-resistant Shiga toxin-producing Escherichia coli: An overview of prevalence and intervention strategies. Zoonoses Public Hlth., 66 (1): 1-13.
Missouri Department of Health and Senior Services (2010). Communicable Disease Surveillance 2010 Annual Report.
Moore, M., and Shelton, S. 2014. Updated guidelines for the control of Legionella in Western Pennsylvania. Allegheny County Health Department Pittsburgh Regional Health Initiative. https://www.rand.org/content/dam/rand/pubs/external_publications/EP60000/EP66197/RAND_EP66197.pdf.
Moore, E.R.B., Tindall, B.J., Martins Dos Santos, V.A.P., Pieper, D.H., Ramos, J-L. and Palleroni, N.J. (2006a). Nonmedical: Pseudomonas. In The Prokaryotes—A handbook on the biology of bacteria. Third edition. Volume 4: Bacteria: Firmicutes, Cyanobacteria. Dworkin, M. et al., eds. Springer, Singapore. pp. 646-703.
Moore, M.R., Pryor, M., Fields, B., Lucas, C., Phelan, M. and Besser, R.E. (2006b). Introduction of monochloramine into a municipal water system: Impact on colonization of buildings by Legionella spp. Appl. Env. Microbiol. 72 (1):378-383.
Moreira, N.A. and Bondelind, M. (2017). Safe drinking water and waterborne outbreaks. J. Water. Health., 15(1): 83-96.
Moreno, Y., Piqueres, P., Alonso, J.L., Jiménez, A., González, A. and Ferrús, M.A. (2007). Survival and viability of Helicobacter pylori after inoculation into chlorinated drinking water. Water Res., 41(15): 3490-3496.
Morgan, D.R., Johnson, P.C., DuPont, H.L., Satterwhite, T.K. and Wood, L.V. (1985). Lack of correlation between known virulence properties of Aeromonas hydrophila and enteropathogenicity for humans. Infect. Immun., 50(1): 62-65.
Mraz, A.L. and Weir, M.H. (2018). Knowledge to predict pathogens: Legionella pneumophila lifecycle critical review Part I Uptake into host cells. Water (Switzerland), 10(2), 132.
Murphy, H.M., Thomas, M.K., Schmidt, P.J., Medeiros, D.T., McFadyen, S. and Pintar, K.D.M. (2016). Estimating the burden of acute gastrointestinal illness due to Giardia, Cryptosporidium, Campylobacter, E. coli O157 and norovirus associated with private wells and small water systems in Canada. Epidemiol. Infect., 144 (7): 1355-1370.
Naja, F., Kreiger, N. and Sullivan, T. (2007). Helicobacter pylori infection in Ontario: Prevalence and risk factors. Can. J. Gastroenterol., 21 (8):501-506.
NASEM. (2020). Management of Legionella in Water Systems. National Academies of Sciences, Engineering, and Medicine. Washington, DC. The National Academies Press. https://doi.org/10.17226/25474.
Neil, K. and Berkelman, R. (2008). Increasing incidence of legionellosis in the United States, 1990-2005: Changing epidemiologic trends. Clin. Infect. Dis., 47(5): 591-599.
Neu, L. and Hammes, F. (2020). Feeding the building plumbing microbiome: The importance of synthetic polymeric materials for biofilm formation and management. Water, 12(64): 1774.
NHMRC, NRMMC. (2011). Australian drinking water guidelines 6—national water quality management strategy. National Health and Medical Research Council, National Resource Management Ministerial Council, Commonwealth of Australia, Canberra.
Nichols, G., Ford, T., Bartram, J., Dufour, A. and Portaels, F. (2004). Introduction. In Pathogenic mycobacteria in water—A guide to public health consequences, monitoring and management. Pedley, S. et al., (eds). World Health Organization, Geneva, Switzerland. pp. 1-14.
Nichols, G., Lake, I. and Heaviside, C. (2018). Climate change and water-related infectious diseases. Atmosphere, 9(10).
Nishiuchi, Y., Iwamoto, T. and Maruyama, F. (2017). Infection sources of a common non-tuberculous mycobacterial pathogen, Mycobacterium avium complex. Front. Med., 4(MAR).
Norton, C.D., LeChevallier, M.W. and Falkinham III, J.O. (2004). Survival of Mycobacterium avium in a model distribution system. Water Res., 38(6): 1457-1466.
NRCC. (2015a). National building code. National Research Council of Canada, Ottawa, Ontario. Available at https://nrc.canada.ca/en/certifications-evaluations-standards/codes-canada/codes-canada-publications/national-building-code-canada-2015
NRCC. (2015b). National plumbing code of Canada. National Research Council of Canada, Ottawa, Ontario. Available at https://nrc.canada.ca/en/certifications-evaluations-standards/codes-canada/codes-canada-publications/national-plumbing-code-canada-2015
NSF/ANSI. (2018). Standard 244—Drinking Water Treatment Units-Supplemental Microbiological Water Treatment Systems-Filtration. NSF International, Ann Arbor, Michigan
NSF/ANSI. (2019). Standard 55—Ultraviolet microbiological water treatment systems. NSF International, Ann Arbor, Michigan.
NSF/ANSI. (2020). Standard 60- Drinking water treatment chemicals: Health effects. NSF International, Ann Arbor, Michigan.
O’Connor, D.R. (2002a). Report of the Walkerton inquiry, Part one: The events of May 2000 and related issues. Ontario Ministry of the Attorney General (ISBN: 0-7794-2559-6).
O’Connor, D.R. (2002b). Report of the Walkerton inquiry, Part two report: A strategy for safe drinking water. Ontario Ministry of the Attorney General (ISBN: 0-7794-2621-5)
Orkis, L.T., Harrison, L.H., Mertz, K.J., Brooks, M.M., Bibby, K.J. and Stout, J.E. (2018). Environmental sources of community-acquired Legionnaires’ disease: A review. Int. J. Hyg. Environ. Heal., 221 (5):764-774.
Orta de Velásquez, M. T., Yáñez Noguez, I., Casasola Rodríguez, B. and Román Román, P.I. (2017). Effects of ozone and chlorine disinfection on VBNC Helicobacter pylori by molecular techniques and FESEM images. Environ. Technol., 38(6): 744-753.
O'Ryan, M., Vidal, R., Del Canto, F., Salazar, J.C. and Montero, D. (2015). Vaccines for viral and bacterial pathogens causing acute gastroenteritis: Part II: Vaccines for Shigella, Salmonella, enterotoxigenic E. coli (ETEC) enterohemorragic E. coli (EHEC) and Campylobacter jejuni. Hum. Vacc. and Immunother., 11(3): 601-619.
Padrnos, L.J., Blair, J.E., Kusne, S., Dicaudo, D.J. and Mikhael, J.R. (2014). Cutaneous legionellosis: Case report and review of the medical literature. Transplant Infect. Dis., 16(2): 307-314.
Palusińska-Szysz, M. and Cendrowska-Pinkosz, M. (2009). Pathogenicity of the family Legionellaceae. Arch. Immunol. Ther. Ex., 57(4): 279-290.
Park, J., Kim, J.S., Kim, S., Shin, E., Oh, K-H., Kim, Y., Kim, C.H., Hwang, M.A., Jin, C.M., Na, K., Lee, J., Cho, E., Kang, B-K., Kwak, H-S., Seong, W.K. and Kim, J. (2018). A waterborne outbreak of multiple diarrhoeagenic Escherichia coli infections associated with drinking water at a school camp. Int. J. Infect. Dis., 66: 45-50.
Park, S.R., Mackay, W.G. and Reid, D.C. (2001). Helicobacter sp. recovered from drinking water biofilm sampled from a water distribution system. Water Res., 35(6): 1624-1626.
Percival, S.L. and Williams, D.W. (2014a). Aeromonas. In Microbiology of waterborne diseases: Microbiological aspects and risks: Second edition. Elsevier Ltd. Oxford, UK. pp. 49-64.
Percival, S.L. and Williams, D.W. (2014b). Campylobacter. In Microbiology of waterborne diseases: Microbiological aspects and risks: Second edition. Elsevier Ltd. Oxford, UK. pp. 65-78.
Percival, S.L. and Williams, D.W. (2014c). Escherichia coli. In Microbiology of waterborne diseases: Microbiological aspects and risks: Second edition. Elsevier Ltd. Oxford, UK. pp. 89-117.
Percival, S.L. and Williams, D.W. (2014d). Helicobacter pylori . In Microbiology of waterborne diseases: Microbiological aspects and risks: Second edition. Elsevier Ltd., Oxford, UK. pp. 119-154.
Percival, S.L. and Williams, D.W. (2014e). Legionella. In Microbiology of waterborne diseases: Microbiological aspects and risks: Second edition. Elsevier Ltd. Oxford, UK. pp. 155-175.
Percival, S.L. and Williams, D.W. (2014f). Mycobacterium. In Microbiology of waterborne diseases. Second edition. Elsevier Ltd., Oxford, UK, pp. 177-207.
Percival, S.L. and Williams, D.W. (2014g). Salmonella. In Microbiology of waterborne diseases: Microbiological aspects and risks: Second edition. Elsevier Ltd. Oxford, UK. pp. 209-222.
Percival, S.L. and Williams, D.W. (2014h). Shigella. In Microbiology of waterborne diseases: Microbiological aspects and risks: Second edition. Elsevier Ltd. Oxford, UK. pp. 223-236.
Percival, S.L. and Williams, D.W. (2014i). Yersinia. In Microbiology of waterborne diseases. Second edition. Elsevier Ltd., Oxford, UK, pp. 249-259.
Petrisek, R. and Hall, J. (2018). Evaluation of a most probable number method for the enumeration of Legionella pneumophila from North American potable and nonpotable water samples. J. Water Health, 16 (1), pp. 57-69.
PHAC. (2010). Pathogen Safety Data Sheets: Infectious Substances – Shigella spp. Public Health Agency of Canada, Ottawa, Canada. Accessed September 2019 from: https://www.canada.ca/en/public-health/services/laboratory-biosafety-biosecurity/pathogen-safety-data-sheets-risk-assessment/shigella.html
PHAC. (2011). Pathogen Safety Data Sheets: Infectious Substances – Salmonella enterica spp. Public Health Agency of Canada, Ottawa, Canada. Accessed September 2019 from: https://www.canada.ca/en/public-health/services/laboratory-biosafety-biosecurity/pathogen-safety-data-sheets-risk-assessment/salmonella-enterica.html
PHAC. (2018a). Canadian antimicrobial resistance surveillance system. Update 2018. Public Health Agency of Canada. Ottawa, Canada. Accessed October 2019 from: https://www.canada.ca/en/services/health/publications/drugs-health-products.html
PHAC. (2018b). FoodNet Canada Annual Report 2016. Public Health Agency of Canada, Ottawa, Canada.
PHAC. (2018c). FoodNet Canada Annual Report 2017. Public Health Agency of Canada, Ottawa, Canada.
PHAC. (2018d). Legionella. Public Health Agency of Canada, Ottawa, Canada. Accessed June 2019 from: https://www.canada.ca/en/public-health/services/infectious-diseases/legionella.html.
PHAC. (2019a). FoodNet Canada Annual Report 2018. Public Health Agency of Canada, Ottawa, Canada.
PHAC. (2019b). Notifiable diseases on-line. Reported cases from 1924 to 2016 in Canada. Public Health Agency of Canada, Ottawa, Canada. Accessed September 2019 from: http://diseases.canada.ca/ndis/charts-list.
Piersimoni, C. and Scarparo, C. (2009). Extrapulmonary infections associated with nontuberculous mycobacteria in immunocompetent persons. Emerg. Infect. Dis., 15(9): 1351-1358.
Posteraro, B., Posteraro, P. and Sanguinetti, M. (2015). Helicobacter pylori. In Molecular medical microbiology: Second edition. Elsevier Ltd, London, UK. pp. 1237-1258.
Prussin, A.J., II, Schwake, D.O. and Marr, L.C. (2017). Ten questions concerning the aerosolization and transmission of Legionella in the built environment. Build. Environ., 123: 684-695.
Pryor, M., Springthorpe, S., Riffard, S., Brooks, T., Huo, Y., Davis, G. and Sattar, S.A. (2004). Investigation of opportunistic pathogens in municipal drinking water under different supply and treatment regimes. Water Sci. Technol., 50(1): 83-90.
Purdue University. (2020). Flushing Plans. Centre for Plumbing Safety, Purdue University. West Lafayette, Indiana. Accessed May 2021 from: https://engineering.purdue.edu/PlumbingSafety/resources/flushing-plans
PWGSC. (2016). MD 15161 – 2013 Control of Legionella in Mechanical Systems. Standard for Building Owners, Design Professionals, and Maintenance Personnel. Public Works and Government Services Canada. Gatineau, Quebec. Accessed June 2019 from: https://www.tpsgc-pwgsc.gc.ca/biens-property/legionella/index-eng.html
Qin, K., Struewing, I., Domingo, J.S., Lytle, D. and Lu, J. (2017). Opportunistic pathogens and microbial communities and their associations with sediment physical parameters in drinking water storage tank sediments. Pathogens, 6(4): 54.
Quilliam, R.S., Williams, A.P., Avery, L.M., Malham, S.K. and Jones, D.L. (2011). Unearthing human pathogens at the agricultural-environment interface: A review of current methods for the detection of Escherichia coli O157 in freshwater ecosystems. Agric. Ecosyst. Environ., 140(3-4): 354-360.
Radomski, N., Betelli, L., Moilleron, R., Haenn, S., Moulin, L., Cambau, E., Rocher, V., Gonçalves, A. and Lucas, F.S. (2011). Mycobacterium behavior in wastewater treatment plant, a bacterial model distinct from Escherichia coli and enterococci. Environ. Sci. Technol., 45(12): 5380-5386.
Ramírez-Castillo, F.Y., Loera-Muro, A., Jacques, M., Garneau, P., Avelar-González, F.J., Harel, J. and Guerrero-Barrera, A.L. (2015). Waterborne pathogens: Detection methods and challenges. Pathogens, 4 (2): 307-334.
Rand, J.L., Gagnon, G.A. and Knowles, A. (2014). Establishing minimum free chlorine residual concentration for microbial control in a municipal drinking water distribution system. Water Pract. Technol., 9(4): 491–501.
Raphael, B.H., Baker, D.J., Nazarian, E., Lapierre, P., Bopp, D., Kozak-Muiznieks, N.A., Morrison, S.S., Lucas, C.E., Mercante, J.W., Musser, K.A. and Winchell, J.M. (2016). Genomic resolution of outbreak-associated Legionella pneumophila serogroup 1 isolates from New York State. Appl. Environ. Microbiol., 82(12): 3582-3590.
Rasheed, S., Hashmi, I. and Campos, L. (2016). Inactivation of Escherichia coli and Salmonellawith chlorine in drinking waters at various pH and temperature levels. Proc. Pak. Acad. Sci., 53(2B): 83-92.
Ratnatunga, C.N., Lutzky, V.P., Kupz, A., Doolan, D.L., Reid, D.W., Field, M., Bell, S.C., Thomson, R.M. and Miles, J.J. (2020). The rise of non-tuberculosis mycobacterial lung disease. Front. Immunol., 11. no. 303.
Reuter, S., Sigge, A., Wiedeck, H. and Trautmann, M. (2002). Analysis of transmission pathways of Pseudomonas aeruginosa between patients and tap water outlets. Crit. Care Med., 30(10): 2222-2228.
Rhoads, W.J., Pruden, A. and Edwards, M.A. (2016). Survey of green building water systems reveals elevated water age and water quality concerns. Environ. Sci.: Wat. Res., 2 (1): 164-173.
Rhoads, W.J., Garner, E., Ji, P., Zhu, N., Parks, J., Schwake, D.O., Pruden, A. and Edwards, M.A. (2017). Distribution system operational deficiencies coincide with reported Legionnaires' disease clusters in Flint, Michigan. Environ. Sci. Technol., 51(20): 11986-11995.
Rice, E.W., Clark, R.M. and Johnson, C.H. (1999). Chlorine inactivation of Escherichia coli O157:H7. Emerg. Infect. Dis., 5(3): 461-463.
Richards, C.L., Broadaway, S.C., Eggers, M.J., Doyle, J., Pyle, B.H., Camper, A.K. and Ford, T.E. (2018). Detection of pathogenic and non-pathogenic bacteria in drinking water and associated biofilms on the Crow Reservation, Montana, USA. Microb. Ecol., 76(1): 52-63.
Robertson, P., Abdelhady, H. and Garduño, R.A. (2014a). The many forms of a pleomorphic bacterial pathogen-the developmental network of Legionella pneumophila. Front. Microbiol. 5, art. no. 670: 1-20.
Robertson, B.K., Harden, C., Selvaraju, S.B., Pradhan, S. and Yadav, J.S. (2014b). Molecular detection, quantification, and toxigenicity profiling of Aeromonas spp. in source- and drinking-water. Open Microbiol. J., 8(1): 32-39.
Robins-Browne, R.M., Holt, K.E., Ingle, D.J., Hocking, D.M., Yang, J. and Tauschek, M. (2016). Are Escherichia coli pathotypes still relevant in the era of whole-genome sequencing? Front. Cell. Infect. Mi., 6 (NOV), art. no. 141.
Rogues, A-M., Boulestreau, H., Lashéras, A., Boyer, A., Gruson, D., Merle, C., Castaing, Y., Bébear, C.M. and Gachie, J-P. (2007). Contribution of tap water to patient colonisation with Pseudomonas aeruginosa in a medical intensive care unit. J. Hosp. Infect., 67(1): 72-78.
Rohr, U., Weber, S., Michel, R., Selenka, F. and Wilhelm, M. (1998). Comparison of free-living amoebae in hot water systems of hospitals with isolates from moist sanitary areas by identifying genera and determining temperature tolerance. Appl. Environ. Microbiol., 64 (5), pp. 1822-1824.
Rose, L.J., Rice, E.W., Hodges, L., Peterson, A. and Arduino, M.J. (2007). Monochloramine inactivation of bacterial select agents. Appl. Environ. Microbiol., 73(10): 3437-3439.
Roser, D.J., Van Den Akker, B., Boase, S., Haas, C.N., Ashbolt, N.J. and Rice, S.A. (2014). Pseudomonas aeruginosa dose response and bathing water infection. Epidemiol. Infect., 142(3): 449-462.
Roth, D.K. and Cornwell, D.A. (2018). DBP impacts from increased chlorine residual requirements. J. Am. Water Works Assoc., 110(2): 13–28.
Sabina, Y., Rahman, A., Ray, R.C. and Montet D. (2011). Yersinia enterocolitica: Mode of transmission, molecular insights of virulence, and pathogenesis of infection. J. Pathog. 2011. Article ID 429069.
Saetta, D., Richard, R., Leyva, C., Westerhoff, P. and Boyer, T.H. (2021). Data-mining methods predict chlorine-residuals in premise plumbing using low-cost sensors. AWWA Wat. Sci. 3(1), e1214.
Sanchez-Vargas, F.M., Abu-El-Haija, M.A. and Gomez-Duarte, O.G. (2011). Salmonella infections: An update on epidemiology, management, and prevention. Travel Med. Infect. Dis., 9(6): 263-277.
Santiago, P., Moreno, Y. and Ferrús, M.A. (2015). Identification of viable Helicobacter pylori in drinking water supplies by cultural and molecular techniques. Helicobacter, 20(4): 252-259.
Sarkar, P. and Gerba, C.P. (2012). Inactivation of Naegleria fowleri by chlorine and ultraviolet light. J. Am. Water Works Assoc., 104(3): 51-52.
Saxena, G., Bharagava, R.N., Kaithwas, G. and Raj, A. (2015). Microbial indicators, pathogens and methods for their monitoring in water environment. J. Water Health, 13(2): 319-339.
Scallan, E., Hoekstra, R. M., Angulo, F. J., Tauxe, R. V., Widdowson, M., Roy, S. L. and Griffin, P. M. (2011). Foodborne Illness Acquired in the United States—Major Pathogens. Emerg. Infect. Dis., 17(1), 7-15. https://dx.doi.org/10.3201/eid1701.p11101.
SCC. (2019). Directory of Accredited Product, Process and Service Certification Bodies. Standards Council of Canada, Ottawa, Ontario. Available at https://www.scc.ca/en/accreditation/product-process-and-service-certification/directory-of-accredited-clients
Schaffter, N. and Parriaux, A. (2002). Pathogenic-bacterial water contamination in mountainous catchments. Water Res., 36(1): 131-139.
Schiavano, G.F., De Santi, M., Sisti, M., Amagliani, G. and Brandi, G. (2018). Disinfection of Mycobacterium avium subspecies hominissuis in drinking tap water using ultraviolet germicidal irradiation. Environ.Technol. (United Kingdom), 39(24): 3221-3227.
Schulze-Robbecke, R. and Buchholtz, K. (1992). Heat susceptibility of aquatic mycobacteria. Appl. Environ. Microbiol., 58(6): 1869-1873.
Schwake, D.O., Garner, E., Strom, O.R., Pruden, A. and Edwards, M.A. (2016). Legionella DNA markers in tap water coincident with a spike in Legionnaires' disease in Flint, MI. Environ. Sci. Technol. Let., 3(9): 311-315.
Sebakova, H., Kozisek, F., Mudra, R., Kaustova, J., Fiedorova, M., Hanslikova, D., Nachtmannova, H., Kubina, J., Vraspir, P. and Sasek, J. (2008). Incidence of nontuberculous mycobacteria in four hot water systems using various types of disinfection. Can. J. Microbiol., 54(11): 891-898.
Segal, I., Otley, A., Issenman, R., Armstrong, D., Espinosa, V., Cawdron, R., Morshed, M.G. and Jacobson, K. (2008). Low prevalence of Helicobacter pylori infection in Canadian children: A cross-sectional analysis. Can. J. Gastroenterol., 22 (5):485-489.
Semenza, J.C. (2020). Cascading risks of waterborne diseases from climate change. Nat. Immunol., 21(5): 484-487.
Sen, K. and Rodgers, M. (2004). Distribution of six virulence factors in Aeromonas species isolated from US drinking water utilities: A PCR identification. J. Appl. Microbiol., 97(5): 1077-1086.
Sethi, A., Chaudhuri, M., Kelly, L. and Hopman, W. (2013). Prevalence of Helicobacter pylori in a First Nations population in northwestern Ontario. Can. Fam. Physician, 59 (4): e182-e187.
Schuster, C.J., Ellis, A.G., Robertson, W.J., Charron, D.F., Aramini, J.J., Marshall, B.J. and Medeiros, D.T. (2005). Infectious disease outbreaks related to drinking water in Canada, 1974-2001. Can. J. Public Health, 96(4): 254-255.
Sharma, S. and Upadhyay, V. (2020). Epidemiology, diagnosis & treatment of non-tuberculous mycobacterial diseases. Indian J. Med. Res., 152(3): 185-226.
Siddiqui, R., Ali, I.K.M., Cope, J.R. and Khan, N.A. (2016). Biology and pathogenesis of Naegleria fowleri. Acta Trop., 164: 375-394.
Singh, R., Hamilton, K.A., Rasheduzzaman, M., Yang, Z., Kar, S., Fasnacht, A., Masters, S.V. and Gurian, P.L. (2020). Managing water quality in premise plumbing: Subject matter experts' perspectives and a systematic review of guidance documents. Water (Switzerland), 12 (2), art. no. 347.
Sisti, M., Albano, A. and Brandi, G. (1998). Bactericidal effect of chlorine on motile Aeromonas spp. in drinking water supplies and influence of temperature on disinfection efficacy. Lett. Appl. Microbiol., 26(5): 347-351.
Smeets, P., Rietveld, L., Hijnen, W., Medema, G. and Stenstrom, T. (2006). Efficacy of water treatment processes. In MicroRisk—microbiological risk assessment: A scientific basis for managing drinking water safety from source to tap. April 2006. Accessed June 2019 from: www.kwrwater.nl
Smeets, P. W. M. H., Medema, G.J. and Van Dijk, J.C. (2009). The Dutch secret: How to provide safe drinking water without chlorine in the Netherlands. Drink. Water Eng. Sci., 2(1): 1-14.
Sobsey, M.D. (1989). Inactivation of health-related microorganisms in water by disinfection processes. Water Sci. Technol., 21(3): 179-195.
Soda, E.A. (2017). Vital signs: Health Care–Associated Legionnaires’ disease surveillance data from 20 states and a large metropolitan area—United States, 2015. MMWR Morb Mortal Wkly Rep, 66.
Solnick, J.V., Hansen, L.M., Canfield, D.R. and Parsonnet, J. (2001). Determination of the infectious dose of Helicobacter pylori during primary and secondary infection in rhesus monkeys ( Macaca mulatta). Infect. Immun., 69(11): 6887-6892.
Sommer, R., Lhotsky, M., Haider, T. and Cabaj, A. (2000). UV inactivation, liquid-holding recovery, and photoreactivation of Escherichia coli O157 and other pathogenic Escherichia coli strains in water. J. Food Prot., 63(8): 1015-1020.
Sood, G. and Parrish, N. (2017). Outbreaks of nontuberculous mycobacteria. Curr. Opin. Infect. Dis., 30(4): 404-409.
Spiegelman, J., Pedutem, T. and Francisco, M.J. (2020). Legionnaires’ disease cases at a large community hospital—common and underdiagnosed. Int. J. Environ. Res. Public Health, 17(1).
Spinale, J.M., Ruebner, R.L., Copelovitch, L. and Kaplan, B.S. (2013). Long-term outcomes of Shiga toxin hemolytic uremic syndrome. Pediatr. Nephrol., 28 (11):2097-2105.
Springston, J.P. and Yocavitch, L. (2017). Existence and control of Legionella bacteria in building water systems: A review. J. Occup. Environ. Hyg., 14(2): 124-134.
Sterk, A., Schijven, J., De Nijs, T. and De Roda Husman, A.M. (2013). Direct and indirect effects of climate change on the risk of infection by water-transmitted pathogens. Environ. Sci. Technol., 47 (22):12648-12660.
Stinear, T., Ford, T. and Vincent, V. (2004). Analytical methods for the detection of waterborne and environmental pathogenic mycobacteria. In Pathogenic mycobacteria in water. A guide to public health consequences, monitoring and management. Pedley, S. (ed.). IWA Publishing. World Health Organization., Geneva, Switzerland. pp. 55-73.
Stout, J.E., YU, V.L., Yee, Y.C., Vaccarello, S., Diven, W. and Lee, T.C. (1992). Legionella pneumophila in residential water supplies: Environmental surveillance with clinical assessment for Legionnaires' disease. Epidemiol. Infect., 109(1): 49-57.
Stout, J.E., Koh, W-J. and Yew, W.W. (2016). Update on pulmonary disease due to non-tuberculous mycobacteria. Int. J. Infect. Dis., 45: 123-134.
Surman-Lee, S., Fields, B., Hornei, B., Ewig, S., Exner, M., Tartakovsky, I., Lajoie, L., Dangendorf, F., Bentham, R., Cabanes, P.A., Fourrier, P., Trouvet, T. and France Wallet, F. (2007). Chapter 2: Ecology and environmental sources of Legionella. In Legionella and the prevention of legionellosis. Bartram, J. et.al. (eds.). World Health Organization, Geneva, Switzerland. pp. 29-38.
Taylor, R.H., Falkinham III, J.O., Norton, C.D. and LeChevallier, M.W. (2000). Chlorine, chloramine, chlorine dioxide, and ozone susceptibility of Mycobacterium avium. Appl. Environ. Microbiol., 66(4): 1702-1705.
Tempark, T., Lueangarun, S., Chatproedprai, S. and Wananukul, S. (2013). Flood-related skin diseases: A literature review. Int. J. Dermatol., 52(10): 1168-1176.
Teunis, P. and Figueras, M.J. (2016). Reassessment of the enteropathogenicity of mesophilic Aeromonas species. Front. Microbiol., 7(Article 1395):1-12.
Teunis, P.F.M., van den Brandhof, W., Nauta, M., Wagenaar, J., van den Kerkhof, H. and van Pelt, W. (2005). A reconsideration of the Campylobacter dose - Response relation. Epidemiol. Infect., 133 (4): 583-592.
Teunis, P.F.M., Bonačić Marinović, A., Tribble, D.R., Porter, C.K. and Swart, A. (2018). Acute illness from Campylobacter jejuni may require high doses while infection occurs at low doses. Epidemics, 24:1-20.
Thomas, M.K., Charron, D.F., Waltner-Toews, D., Schuster, C., Maarouf, A.R. and Holt, J.D. (2006). A role of high impact weather events in waterborne disease outbreaks in Canada, 1975-2001. Int. J. Environ. Heal. R., 16 (3): 167-180.
Thomas, J.M. and Ashbolt, N.J. (2011). Do free-living amoebae in treated drinking water systems present an emerging health risk? Environ. Sci. Technol., 45(3): 860-869.
Thomson, R., Carter, R., Gilpin, C., Coulter, C. and Hargreaves, M. (2008) Comparison of methods for processing drinking water samples for the isolation of Mycobacterium avium and Mycobacterium intracellulare. Appl. Environ. Microbiol., 74, 3094–3098.
Thomson, R.M., Carter, R., Tolson, C., Coulter, C., Huygens, F. and Hargreaves, M. (2013). Factors associated with the isolation of nontuberculous mycobacteria (NTM) from a large municipal water system in Brisbane, Australia. BMC Microbiol., 13(1).
Todd, E.C.D. (2014). Bacteria: Yersinia enterocolitica and Yersinia pseudotuberculosis. In Encyclopedia of food safety. Volume 1. Academic Press, Cambridge, USA, pp. 574-580.
Tossa, P., Deloge-Abarkan, M., Zmirou-Navier, D., Hartemann, P. and Mathieu, L. (2006). Pontiac fever: An operational definition for epidemiological studies. BMC Public Health, 6(1): 112.
Tyrrel, S.F. and Quinton, J.N. (2003). Overland flow transport of pathogens from agricultural land receiving faecal wastes. J. Appl. Microbiol. Symp. Suppl., 94(32): 87S-93S.
US EPA. (1989). National Primary Drinking Water Regulations; Filtration, Disinfection; Turbidity, Giardia lamblia, Viruses, Legionella, and Heterotrophic Bacteria; Final Rule. Part III. Federal Register, 54:124:27486. (June 29, 1989).
US EPA. (2001). Method 1605: Aeromonas in Finished Water by Membrane Filtration using Ampicillin-Dextrin Agar with Vancomycin (ADA-V). United States Environmental Protection Agency, Office of Water. Washington, DC. EPA-821-R-01-034.
US EPA. (2006a). Aeromonas: Human Health Criteria Document. United States Environmental Protection Agency, Office of Water. Washington, D.C. March 2006.
US EPA. (2006b). National primary drinking water regulations: Long term 2 enhanced surface water treatment rule. Final rule. Fed. regist., 71(3): 653–702.
US EPA. (2016). Technologies for Legionella control in premise plumbing systems: Scientific literature review. United States Environmental Protection Agency, Office of Water. Washington, DC. EPA 810-R-16-001. September 2016.
US EPA (2021a). Drinking water regulations. Drinking water requirements for states and public water systems. United States Environmental Protection Agency, Office of Water. Washington, DC. Accessed August 2021 from https://www.epa.gov/dwreginfo/drinking-water-regulations
US EPA. (2021b). Public Engagements: Potential Revisions of the Microbial and Disinfection Byproducts Rules. United States Environmental Protection Agency, Office of Water. Washington, DC. Accessed August 2021 from: https://www.epa.gov/dwsixyearreview/public-engagements-potential-revisions-microbial-and-disinfection-byproducts-rules
van der Wielen, P. W. J. J. and van der Kooij, D. (2013). Nontuberculous mycobacteria, fungi, and opportunistic pathogens in unchlorinated drinking water in the Netherlands. Appl. Environ. Microbiol., 79(3): 825-834.
Vicuña-Reyes, J.P., Luh, J. and Mariñas, B.J. (2008). Inactivation of Mycobacterium avium with chlorine dioxide. Water Res., 42(6-7): 1531-1538.
Villegas, P. (2020). Brain-eating amoeba in city’s water supply kills 6-year-old, leads Texas to declare a disaster. The Washington Post. https://www.washingtonpost.com/
Vinnard, C., Longworth, S., Mezochow, A., Patrawalla, A., Kreiswirth, B.N. and Hamilton, K. (2016). Deaths related to nontuberculous mycobacterial infections in the United States, 1999-2014. Annals of the American Thoracic Society, 13(11): 1951-1955.
Visvesvara, G.S., Moura, H. and Schuster, F.L. (2007). Pathogenic and opportunistic free-living amoebae: Acanthamoeba spp., Balamuthia mandrillaris, Naegleria fowleri, and Sappinia diploidea. FEMS Immunol. Med. Mic., 50(1): 1-26.
von Reyn, C.F., Pozniak, A., Haas, W. and Nichols, G. (2004). Disseminated infection, cervical adenitis and other MAC infections. In Pathogenic mycobacteria in water—A guide to public health consequences, monitoring and management. Pedley, S. et al., (eds). World Health Organization, Geneva, Switzerland. pp: 95-103.
Waak, M.B., LaPara, T.M., Hallé, C. and Hozalski, R.M. (2018). Occurrence of Legionella spp. in water-main biofilms from two drinking water distribution systems. Environ. Sci. Technol., 52(14): 7630–7639.
Waddell, L.A., Rajic, A., Stärk, K.D.C. and McEwen, S.A. (2015). The zoonotic potential of Mycobacterium avium ssp. paratuberculosis: A systematic review and meta-analyses of the evidence. Epidemiol. Infect., 143 (15):3135-3157.
Waddell, L., Rajić, A., Stärk, K. and McEwen, S.A. (2016). Mycobacterium avium ssp. paratuberculosis detection in animals, food, water and other sources or vehicles of human exposure: A scoping review of the existing evidence. Prev. Vet. Med., 132:32-48.
Wagenaar, J.A., Newell, D.G., Kalupahana, R.S. and Lapo Mughini-Gras, L. (2015). Campylobacter: Animal reservoirs, human infections, and options for control. In Zoonoses-infections affecting humans and animals: Focus on public health aspects. Sing, A. (ed.). Springer. Dordrecht, Netherlands. pp. 159-177.
Walker, J.T. (2018). The influence of climate change on waterborne disease and Legionella: A review. Perspect. Public Health, 138(5): 282-286.
Walser, S.M., Gerstner, D.G., Brenner, B., Höller, C., Liebl, B. and Herr, C.E.W (2014). Assessing the environmental health relevance of cooling towers - A systematic review of legionellosis outbreaks. Int. J. Hyg. Environ. Heal., 217 (2-3):145-154.
Wang, H., Edwards, M., Falkinham, J.O. and Pruden, A. (2012a). Molecular survey of the occurrence of Legionella spp., Mycobacterium spp., Pseudomonas aeruginosa, and amoeba hosts in two chloraminated drinking water distribution systems. Appl. Environ. Microbiol., 78(17): 6285-6294.
Wang, H., Masters, S., Hong, Y., Stallings, J., Falkinham, J.O.,3rd, Edwards, M.A. and Pruden, A. (2012b). Effect of disinfectant, water age, and pipe material on occurrence and persistence of Legionella, mycobacteria, Pseudomonas aeruginosa, and two amoebas. Environ. Sci. Technol., 46(21): 11566-11574.
Wang, H., Bédard, E., Prévost, M., Camper, A.K., Hill, V.R. and Pruden, A. (2017). Methodological approaches for monitoring opportunistic pathogens in premise plumbing: A review. Water Res., 117: 68-86.
Watson, C.L., Owen, R.J., Said, B., Lai, S., Lee, J.V., Surman-Lee, S. and Nichols, G. (2004). Detection of Helicobacter pylori by PCR but not culture in water and biofilm samples from drinking water distribution systems in England. J. Appl. Microbiol., 97(4): 690-698.
Weigel, K.M., Nguyen, F.K., Kearney, M.R., Meschke, J.S. and Cangelosi, G.A. (2017). Molecular viability testing of UV-inactivated bacteria. Appl. Environ. Microbiol., 83(10).
Weiss, D., Boyd, C., Rakeman, J.L., Greene, S.K., Fitzhenry, R., McProud, T., … and Varma, J.K. (2017). A large community outbreak of Legionnaires’ disease associated with a cooling tower in New York City, 2015. Public Health Rep., 132(2): 241-250.
Whiley, H., Keegan, A., Giglio, S. and Bentham, R. (2012). Mycobacterium avium complex - the role of potable water in disease transmission. J. Appl. Microbiol., 113(2): 223-232.
Whiley, H., van den Akker, B., Giglio, S. and Bentham, R. (2013). The role of environmental reservoirs in human campylobacteriosis. Int. J. Environ. Res. Public Health, 10(11): 5886-5907.
Whiley, H., Keegan, A., Fallowfield, H. and Bentham, R. (2014). Detection of Legionella, L. pneumophila and Mycobacterium avium complex (MAC) along potable water distribution pipelines. Int. J. Env. Res. Pub. He., 11(7): 7393-7405.
WHO. (2002). Guidelines for drinking-water quality Second Edition. Addendum: Microbiological agents in drinking water. World Health Organization, Geneva, Switzerland.
WHO. (2004). Pathogenic Mycobacteria in Water—A Guide to Public Health Consequences, Monitoring and Management. Pedley, S. et al., (eds). World Health Organization, Geneva, Switzerland.
WHO. (2007). Legionella and the prevention of legionellosis. Bartram, J. (ed.). World Health Organization, Geneva, Switzerland.
WHO. (2011). Water safety in buildings. World Health Organization. Geneva, Switzerland.
WHO (2017a). Guidelines for drinking-water quality: fourth edition incorporating the first addendum.
Geneva: World Health Organization; 2017.
WHO. (2017b). Prioritization of pathogens to guide discovery, research and development of new antibiotics for drug-resistant bacterial infections, including tuberculosis. World Health Organization. Geneva, Switzerland. Accessed October 2019 from https://www.who.int/medicines/areas/rational_use/prioritization-of-pathogens/en/
WHO and OECD (2003). Assessing Microbial Safety of Drinking Water – Improving approaches and methods. World Health Organization and Organisation for Economic Co-operation and Development. IWA Publishing, London UK. pp 1-295.
Williams, M.M., Chen, T., Keane, T., Toney, N., Toney, S., Armbruster, C.R., Ray Butler, R. and Arduino, M.J. (2011). Point-of-use membrane filtration and hyperchlorination to prevent patient exposure to rapidly growing mycobacteria in the potable water supply of a skilled nursing facility. Infect. Con. Hosp. Ep., 32(9): 837-844.
Wilson, J., Aramini, J., Clarke, S., Novotny, M., Quist, M. and Keegan, V. (2009). Retrospective surveillance for drinking water-related illnesses in Canada, 1993-2008: Final report. Novometrix Research Inc. Moffat, Ontario. Accessed July 2021 from: https://www.ncceh.ca/sites/default/files/DW_Illnesses_Surveillance_Aug_2009.pdf
Wilson, R.E., Hill, R.L.R., Chalker, V.J., Mentasti, M., and Ready, D. (2018). Antibiotic susceptibility of Legionella pneumophila strains isolated in England and Wales 2007–17. J. Antimicrob. Chemoth., 73 (10): 2757-2761.
Wingender, J. and Flemming, H-C. (2004). Contamination potential of drinking water distribution network biofilms. Water Sci. Technol., 49(11-12): 277-286.
Winiecka-Krusnell, J., Wreiber, K., Von Euler, A., Engstrand, L. and Linder, E. (2002). Free-living amoebae promote growth and survival of Helicobacter pylori. Scand. J. Infect. Dis., 34(4): 253-256.
Wojcicka, L., Hofmann, R., Baxter, C., Andrews, R.C., Auvray, I., Lière, J., Miller, T., Chauret, C. and Baribeau, H. (2007). Inactivation of environmental and reference strains of heterotrophic bacteria and Escherichia coli O157:H7 by free chlorine and monochloramine. J. Water Supply Res. Technol. Aqua, 56(2): 137-150.
Wong, E.A. and Shin, G-A. (2015). Removal of Mycobacterium avium subspecies hominissuis (MAH) from drinking water by coagulation, flocculation and sedimentation processes. Lett. Appl. Microbiol., 60(3): 273-278.
Xue, Z., Hessler, C.M., Panmanee, W., Hassett, D.J. and Seo, Y. (2013). Pseudomonas aeruginosa inactivation mechanism is affected by capsular extracellular polymeric substances reactivity with chlorine and monochloramine. FEMS Microbiol. Ecol., 83(1): 101-111.
Yoder, J., Roberts, V., Craun, G.F., Hill, V., Hicks, L.A., Alexander, N.T., Radke, V., Calderon, R.L., Hlavsa, M.C., Beach, M.J. and Roy, S.L. (2008). Surveillance for waterborne disease and outbreaks associated with drinking water and water not intended for drinking—United States, 2005-2006. MMWR Surveill. Summ., 57(9): 39-62.
Yoder, J.S., Eddy, B.A., Visvesvara, G.S., Capewell, L. and Beach, M.J. (2010). The epidemiology of primary amoebic meningoencephalitis in the USA, 1962-2008. Epidemiol. Infect., 138(7): 968-975.
Yoder, J.S., Straif-Bourgeois, S., Roy, S.L., Moore, T.A., Visvesvara, G.S., Ratard, R.C., Hill, V.R., Wilson, J.D., Linscott, A.J., Crager, R., Kozak, N.A., Sriram, R., Narayanan, J., Mull, B., Kahler, A.M., Schneeberger, C., Da Silva, A.J., Poudel, M., Baumgarten, K.L., Xiao, L. and Beach, M.J. (2012a). Primary amebic meningoencephalitis deaths associated with sinus irrigation using contaminated tap water. Clin. Infect. Dis., 55(9): e7-e85.
Yoder, J.S., Verani, J., Heidman, N., Hoppe-Bauer, J., Alfonso, E.C., Miller, D., Jones, D.B., Bruckner, D., Langston, R., Jeng, B.H., Joslin, C.E., Tu, E., Colby, K., Vetter, E., Ritterband, D., Mathers, W., Kowalski, R.P., Acharya, N.R., Limaye, A.P., Leiter, C., Roy, S., Lorick, S., Roberts, J. and Beach, M.J. (2012b). Acanthamoeba keratitis: The persistence of cases following a multistate outbreak. Ophthalmic Epidemiology, 19(4): 221-225.
Yu, C.-P., Farrell, S.K., Robinson, B. and Chu, K.-H. (2008). Development and application of real-time PCR assays for quantifying total and aerolysin gene-containing Aeromonas in source, intermediate, and finished drinking water. Environ. Sci. Technol., 42(4): 1191-1200.
Zahran, S., McElmurry, S.P., Kilgore, P.E., Mushinski, D., Press, J., Love, N.G., Sadler, R.C. and Swanson, M.S. (2018). Assessment of the Legionnaires’ disease outbreak in Flint, Michigan. Proceedings of the National Academy of Sciences of the United States of America, 115(8): E1730-E1739.
Zamani, M., Ebrahimtabar, F., Zamani, V., Miller, W.H., Alizadeh-Navaei, R., Shokri-Shirvani, J. and Derakhshan, M.H. (2018). Systematic review with meta-analysis: the worldwide prevalence of Helicobacter pylori infection. Aliment. Pharm. Therap., 47 (7):868-876.
Zaongo, S.D., Shaio, M.-F. and Ji, D.-D. (2018). Effects of culture media on Naegleria fowleri growth at different temperatures. J. Parasitol., 104 (5):451-456.
Zautner, A.E., Masanta, W.O. (2016). Campylobacter: Health Effects and Toxicity. In Encyclopedia of Food and Health. Caballero, B. et al. (eds.). Academic Press, Cambridge, USA. pp. 596-601.
Zhang, Y.Q., Wu, Q.P., Zhang, J.M., Yang, X.H. (2015). Effects of ozone on the cytomembrane and ultrastructure of Pseudomonas aeruginosa. Food Sci. Biotechnol., 24(3): 987-993.
Zimmer, J.L. and Slawson, R.M. (2002). Potential repair of Escherichia coli DNA following exposure to UV radiation from both medium- and low-pressure UV sources used in drinking water treatment. Appl. Environ. Microbiol., 68 (7): 3293-3299.
Zimmer-Thomas, J.L., Slawson, R.M. and Huck, P.M. (2007). A comparison of DNA repair and survival of Escherichia coli O157:H7 following exposure to both low- and medium- pressure UV irradiation. J. Water Health, 5(3): 407-415.
Zuma, F.N., Lin, J. and Jonnalagadda, S.B. (2009). Kinetics of inactivation of Pseudomonas aeruginosa in aqueous solutions by ozone aeration. J. Environ. Sci. Health Part A Toxic Hazard. Subst. Environ. Eng., 44(10): 929-935.
Part D: Appendices
Appendix A: List of Abbreviations
- AIDS
- acquired immunodeficiency syndrome
- AK
- Acanthamoeba keratitis
- ANSI
- American National Standards Institute
- ASHRAE
- American Society of Heating, Refrigerating, and Air-Conditioning Engineers
- CDC
- Centers for Disease Control and Prevention
- CFU
- colony forming units
- CT
- concentration (C) × time (T)
- DAEC
- diffuse adherent Escherichia coli
- DNA
- deoxyribonucleic acid
- EAEC
- enteroaggregative Escherichia coli
- E. coli
- Escherichia coli
- EHEC
- enterohaemorrhagic Escherichia coli
- EIEC
- enteroinvasive Escherichia coli
- EPEC
- enteropathogenic Escherichia coli
- ESBL
- extended spectrum β-lactamase
- ETEC
- enterotoxigenic Escherichia coli
- EU
- European Union
- GAC
- granulated activated carbon
- GAE
- granulomatous amoebic encephalitis
- HIV
- human immunodeficiency virus
- HPC
- heterotrophic plate count
- HUS
- hemolytic uremic syndrome
- HVAC
- heating, ventilation and air conditioning
- IARC
- International Agency for Research on Cancer
- ISO
- International Organization for Standardization
- NASEM
- National Academies of Sciences, Engineering and Medicine
- NPC
- National Plumbing Code (Canada)
- NSF
- NSF International
- NTM
- non-tuberculous mycobacteria
- PAM
- primary amebic meningoencephalitis
- PCR
- polymerase chain reaction
- PHAC
- Public Health Agency of Canada
- POE
- point-of-entry
- POU
- point-of-use
- QMRA
- quantitative microbial risk assessment
- SCC
- Standards Council of Canada
- spp.
- species
- US EPA
- United States Environmental Protection Agency
- U.S.
- United States
- UV
- ultraviolet
- VBNC
- viable but non-culturable
- VTEC
- verotoxin-producing Escherichia coli
- WHO
- World Health Organization
Pathogen | Members most frequently associated with human illness | Health Effects | Groups at higher risk for illness | Main reservoirs | Major route for waterborne transmission | Significance as a drinking water pathogen |
---|---|---|---|---|---|---|
Campylobacter spp. | C. jejuni, C. coli | Gastroenteritis (symptoms: watery, profuse and sometimes bloody diarrhea; fever and abdominal pain). | Young children, young adults, the elderly. | Poultry, cattle, sheep, domestic pets. | Ingestion of fecally contaminated water. | Well-documented as a cause of outbreaks associated with contaminated drinking water. |
Pathogenic Escherichia coli/Shigella spp. | Enterohaemorrhagic E. coli (EHEC) group. E. coli serotype O157:H7 is the most prevalent. | Gastroenteritis (symptoms: watery diarrhea that can be accompanied by blood, nausea, vomiting, abdominal pain, fever). EHEC illness can progress to the hemolytic uremic syndrome (HUS), a potentially life-threatening condition involving decreased blood cell and platelet counts and acute kidney failure. |
Young children, the elderly. | Cattle and other ruminants, humans. | Ingestion of fecally-contaminated drinking water. | Well-documented as a cause of outbreaks associated with contaminated drinking water. |
Shigella spp.: S. sonnei and S. flexneri | Gastroenteritis (symptoms: watery diarrhea that can be accompanied by blood, abdominal pain, fever). | Young children. | Humans. | Ingestion of fecally-contaminated water. | Rarely linked to drinking water outbreaks | |
Helicobacter pylori | H. pylori | Asymptomatic superficial gastritis. Some infections develop into peptic (e.g., duodenal or gastric) ulcers. | Individuals living in areas with crowded or high density living conditions and/or poor sanitation. | Humans. | Ingestion of fecally-contaminated water suspected as a possible route. | Further research is needed on the importance of drinking water as a source of infection. |
Salmonella spp. | Non-typhoidal Salmonella groupFootnote a, particularly: S. serotype Enteritidis and S. serotype Typhimurium. | Gastroenteritis (diarrhea, fever and abdominal pain). Severe cases with immunocompromised individuals: can spread to other parts of the body (e.g., blood, urine, joints, brain) |
Young children, the elderly, persons with weakened immune systems. | Chicken, pigs, turkey and cattle. | Ingestion of fecally-contaminated water. | Rarely linked to drinking water outbreaks. |
Yersinia spp. | Y. enterocolitica, Y. paratuberculosis | Gastroenteritis ranging in severity depending on the strain (symptoms: diarrhea that can be accompanied by blood, fever and abdominal pain in children; appendicitis-like symptoms in adults). | Young children, the elderly, persons with weakened immune systems. | Y. enterocolitica: Pigs, ruminants, dogs, cats. Y. paratuberculosis: rodents, birds |
Ingestion of fecally-contaminated water. | Rarely linked to drinking water outbreaks. |
|
Pathogen | Members most frequently associated with human illness | Health Effects | Groups at higher risk for illness. | Main reservoirs | Major route for waterborne transmission | Significance as a drinking water pathogen |
---|---|---|---|---|---|---|
Aeromonas spp. | A. hydrophila, A. caviae, A. veronii (biotype sobria), A. trota | Linked to a variety of intestinal and extra-intestinal diseases and syndromes. Gastroenteritis is the most common disease (symptoms: watery diarrhea along with low grade fever, vomiting and abdominal pain). |
Young children, the elderly, persons with weakened immune systems. | Ubiquitous bacteria, found in a wide variety of habitats, including aquatic habitats, soils, vertebrate and invertebrate animal species, insects and foods. | Ingestion of contaminated water. | Further research is needed on the importance of drinking water as a source of infection. |
Legionella spp. | L. pneumophila (mainly serogroup 1) | Legionnaires’ disease: severe respiratory illness involving pneumonia. Pontiac Fever: milder, flu-like, self-limiting and non-pneumonic disease. Other disease (extremely rare): extrapulmonary infections involving the skin or soft tissues. |
Older adults beginning at age 40-50, smokers, persons with weakened immune systems or underlying disease. | Free-living protozoa that can be found within biofilms in the natural environment and in engineered water systems and equipment (large plumbing systems, cooling towers, drinking water distribution systems). | Inhalation of contaminated aerosols generated by fittings and equipment associated with plumbing systems, HVAC systems, and humidification equipment. | Well-documented as a cause of outbreaks associated with water systems (cooling towers, plumbing systems) of large buildings (most commonly hospitals, long-term care facilities, hotels and resorts). |
Mycobacterium spp. | Non-tuberculous mycobacteria (NTM), group, particularly: |
Pulmonary disease. Symptoms: persistent cough, weakness and night sweats. Severe cases with immunocompromised individuals, infection can spread to other parts of the body (e.g., joints, liver, brain). Other diseases: cervical lymphadenitis, skin and soft tissue infections. |
Individuals with weakened immune systems or underlying respiratory conditions. | Soils, water habitats, biofilms in engineered water systems (plumbing systems, drinking water distribution systems). | Inhalation of contaminated aerosols generated by fittings and equipment associated with plumbing systems and humidification equipment. | No reported outbreaks associated with drinking water. Contaminated water can be an important source for infections in specific settings (e.g., health care facilities) for at risk groups. |
Pseudomonas spp. | P. aeruginosa | Respiratory infections (symptoms: fever, chills, cough and laboured breathing); infections involving the skin, eyes, ears and urinary tract. | Individuals with weakened immune systems or underlying diseases (particularly cystic fibrosis), patients undergoing procedures with invasive medical devices or have burns or penetrating trauma (surgical incisions, wounds). | Ubiquitous bacteria found in a wide variety of habitats, including soil, aquatic environments, vegetation and biofilms in engineered water systems (plumbing systems). | Direct body contact with contaminated water or medical devices in contact with contaminated water from engineered water systems. | No reported outbreaks associated with drinking water. Contaminated water can be an important source for infections in specific settings (e.g., health care facilities) for at risk groups. |
Acanthamoeba spp. | Acanthamoeba genotype T4 | Acanthamoeba keratitis (AK), a vision-threatening disease (symptoms: blurred vision, intense pain and photosensitivity; later, severe cases, ulceration, swelling, glaucoma, cataracts and blindness). | Contact lens wearers. | Ubiquitous in soil and water; also present in biofilms in engineered water systems and equipment (plumbing systems, drinking water distribution systems cooling towers) and in airborne dust. | Eye contact with lenses exposed to water containing the organisms during lens washing, storage or wear. | Incidence of disease is rare. Can act as hosts for pathogenic bacteria including Legionella and non-tuberculous mycobacteria. |
Naegleria spp. | N. fowleri | Primary Amebic Meningoencephalitis (symptoms similar to viral or bacterial meningitis: headache, fever, nausea and vomiting; later: stiff neck, altered mental status, occasional hallucinations seizures, coma). Infections almost always fatal. | Children and young adults engaging in recreational water activities where organism is prevalent; individuals performing nasal cleansing with non-sterile water. | Warm freshwater environments (lakes, rivers, hot springs) and soils. Can adapt to growth in biofilms in distribution and plumbing systems if conditions are favourable (optimal growth temperature, absence of disinfectant). |
Intranasal contact with contaminated water through diving, swimming, bathing, splashing or nasal cleansing. | Incidence of disease is rare. Infections and isolations from piped water systems have primarily occurred in areas with a subtropical climate. Can act as hosts for pathogenic bacteria including Legionella and non-tuberculous mycobacteria. |
Text description
1Massa et al., 1999, Gerba et al., 2003
2Perez-Recuerda et al., 1998; Xue et al., 2013
3Hoff, 1986; Lund et al., 1996; Johnson et al., 1997; Perez-Recuerda et al., 1998; Rice et al., 1999; Baker et al., 2002; Wojcicka et al., 2007; Rasheed et al., 2016
4Health Canada, 2019c
5Buse et al., 2019
6Sakar and Gerba, 2012
7Health Canada, 2019b
8Taylor et al., 2000
9Loret et al., 2008
10Health Canada, 2019b
*3-4 log removal
**4-log removal
***3-log removal
A chart showing the CT values required for achieving a 2 log reduction in numbers for various waterborne pathogens using free chlorine. The x-axis on the chart is the CT values expressed as milligram minutes per Litre. It is on the logarithmic scale and the axis values range from 0.001 to 10000. The y-axis displays different pathogens. Each pathogen is marked with a footnote that corresponds to the references cited. In the chart, the CT values are displayed as bars that extend from the lowest value to the highest value. At the top of the y-axis, the typical design CTs that are required to achieve 4 log virus inactivation and 0.5 log Giardia inactivation are displayed. The CT values displayed are 12-15 and 35-65, respectively. The CT values displayed for the pathogens are as follows: Aeromonas speciese 0.2 to 1.4; Pseudomonas species: 0.0073 to 4.3; E. coli, Campylobacter, Helicobacter, Salmonella, E. coli O157:H7 and Yersinia: 0.034 to 5.1; Enteric viruses: 0.01 to 12; Legionella pneumophila: 0.1 to 0.3; Naegleria fowleri cysts: 31 to 37; Giardia cysts: 25 to 99; Mycobacterium avium: 51 to 1552; Acanthamoeba species cysts: 1300; Cryptosporidium oocysts: Not effective – ensure filtration is optimized or use an alternate primary disinfectant. There is a footnote for the bar for Legionella pneumophila indicating that these values are for a 3 to 4 log removal. There is a footnote for the bar for Naegleria fowleri cysts indicating that these values are for a 4 log removal. There is a footnote for the bar for Mycobacterium avium to indicate that these values are for a 3 log removal. The footnotes below the chart list the references cited.
Text description
1Clauβ et al., 2006
2Gerba et al., 2003
3Health Canada, 2019b
4Health Canada, 2019b
5Hijnen et al., 2011
6Zimmer and Slawson, 2002; Hayes et al., 2006; Zimmer-Thomas et al., 2007; Hijnen et al., 2011
7Health Canada, 2019c
8Hayes et al., 2008; Schiavano et al., 2018
9Sakar and Gerba, 2012
10Hijnen et al., 2011
11Gerba et al., 2003, Schiavano et al., 2018
12Health Canada, 2019c
*2 log removal
**2-5 log removal
A chart showing the UV dose requirements for achieving a 4 log reduction for various waterborne pathogens. The x-axis on the chart is the UV dose expressed as millijoules per square centimetre. It is on the logarithmic scale and the axis values range from 1 to 1000. The y-axis displays different pathogens. Each pathogen is marked with a footnote that corresponds to the references cited. In the chart, the UV doses are displayed as bars that extend from the lowest value to the highest value. The UV doses displayed for the pathogens are: Pseudomonas species: 3.1; Aeromonas species.: 2.5 to 8; Cryptosporidium oocysts: 22; Giardia cysts: 22; Legionella pneumophila: 11 to 30; E. coli, Campylobacter, Helicobacter, Salmonella, E. coli O157:H7 and Yersinia: 5 to 51; Hepatitis A, Coxsackievirus, Poliovirus, Rotavirus: 16.4 to 61; Mycobacterium avium: 12.3 to 64; Naegleria fowleri cysts: 121; Acanthamoeba spp. cysts: 167; Some strains of Mycobacterium species: 96 to192; Adenovirus: 51 to 261. The chart has a footnote markers beside the bar for Aeromonas species to show that these values are for a 2 log removalé The chart has a footnote beside the bar for Some strains of Mycobacterium species to show that these values are for a 2 to 5 log removal. The footnotes below the chart list the references cited.
Page details
- Date modified: