Guidelines for Canadian Drinking Water Quality: Guideline Technical Document – Aluminum
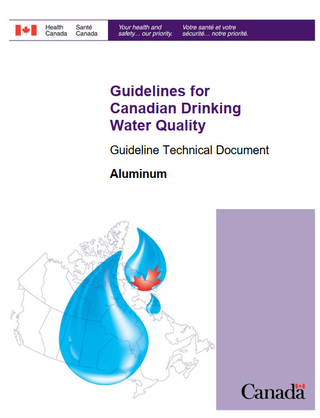
Download the alternative format
(PDF format, 1.8 MB, 72 pages)
Organization: Health Canada
Type: Guidelines
Published: March 2021
Cat: H144-13/18-2021E-PDF
ISBN: 978-0-660-37115-3
Pub: 200347
Related Topics
Table of Contents
- Guideline value
- Executive summary
- Application of the guideline
- International considerations
- 1. Exposure considerations
- 2. Health considerations
- 3. Derivation of the health-based value
- 4. Analytical and Treatment considerations
- 5. Management strategies
- 6. International considerations
- 7. Rationale
- 8. References
- Appendix A: List of abbreviations
- Appendix B: Canadian water quality data
Guideline value
The maximum acceptable concentration (MAC) for total aluminum in drinking water is 2.9 mg/L (2 900 μg/L) based on a locational running annual average of a minimum of quarterly samples taken in the distribution system.
The operational guidance (OG) value for total aluminum in drinking water is 0.100 mg/L (100 μg/L) to optimize water treatment and distribution system operations. This value is based on a locational running annual average. The sampling frequency required to calculate the locational running annual average will vary based on the type of treatment facility and the sampling location.
Other considerations
Strict pH control and adequate coagulant dosing are necessary to optimize coagulation and minimize aluminum residual concentrations. Coagulant under-dosing results in substantial deterioration of pathogen removal capability and increases residual aluminum concentrations. Any strategy used to minimize residual aluminum concentrations must not compromise the removal of pathogens or interfere with the removal of disinfection by-product precursors.
Executive summary
This guideline technical document was prepared in collaboration with the Federal-Provincial-Territorial Committee on Drinking Water and assesses all available information on aluminum.
Exposure
Aluminum is a metal widely distributed in nature. It may be present in water from natural sources or as a result of human activities. The metal is used for many purposes: in the production of construction materials, vehicles, aircraft and electronics; in pharmaceuticals and personal care products; as food additive; and as a component of food packaging materials. Aluminum salts are commonly added as coagulants during water treatment to remove turbidity, organic matter and microorganisms. Aluminum is also an impurity found in other water treatment chemicals and can leach into drinking water from cement-based materials. In addition, aluminum can be added to drinking water as a result of using activated alumina treatment to remove other contaminants (e.g., arsenic, fluoride).
The Canadian population is exposed to aluminum from its presence in the environment and from a variety of products and processes. The main source for Canadians' exposure is through food, followed sequentially by exposure through soil, drinking water and air. Aluminum concentrations in water vary across Canada, with surface water generally presenting higher concentrations than groundwater. Intake of aluminum from drinking water is not expected to occur through either skin contact or inhalation.
Health effects
Aluminum is not an essential element. Studies in humans have found possible associations between aluminum ingestion and diseases of the nervous system. However, these studies have a number of design limitations and do not provide strong evidence that aluminum can cause these diseases. Studies in animals have consistently observed adverse effects on the nervous system following ingestion of high levels of aluminum, which supports effects seen in human studies. The MAC of 2.9 mg/L is based on neurological effects observed in rats.
Operational and aesthetic considerations
Aluminum can act as an accumulation sink for other contaminants such as arsenic, chromium, manganese and nickel and can influence the concentrations of lead and copper. The OG value of 0.100 mg/L applies to their entry points to minimize the potential accumulation and release of aluminum and co-occurring contaminants. Aluminum can also coat watermains, service lines and water meters, resulting in pressure losses, meter malfunctions or turbid/discoloured water. The OG value of 0.100 mg/L is intended to avoid these issues as well.
Analytical and treatment considerations
Several methods are available for analyzing total aluminum in drinking water at concentrations well below the MAC and OG. Online or portable colorimetric analyzers are important tools for obtaining a rapid indication of changes in aluminum concentrations. These measurements can be used to make quick treatment adjustments, which are critical for effective plant operation. Water utilities should confirm with the responsible drinking water authority in the affected jurisdiction whether results from these units can be used for compliance reporting.
Water treatment strategies should minimize the aluminum concentration that enters the distribution system from the treatment plant. For water treatment plants using aluminum-based coagulants, the aluminum residual is an important process parameter (like pH, temperature, turbidity and other measurements) to practice optimum coagulation.
Measures should also be in place to minimize the contribution of aluminum from other water treatment chemicals or processes.
For naturally occurring aluminum in source water, the only known effective treatment technology is coagulation, which is not typically undertaken in small systems or private water supplies. In cases where aluminum removal is required and coagulation is not feasible, the responsible drinking water authority in the affected jurisdiction should be contacted to discuss possible options.
Distribution system
It is recommended that water utilities develop a distribution system management plan to minimize the accumulation and release of aluminum and co-occurring contaminants in the system. This typically involves minimizing the aluminum concentration entering the distribution system and implementing best practices to maintain stable chemical and biological water quality conditions throughout the system, as well as to minimize physical and hydraulic disturbances.
Application of the guidelines
Note: Specific guidance related to the implementation of drinking water guidelines should be obtained from the appropriate drinking water authority.
Due to the effect of pH, temperature and natural organic matter (NOM) on aluminum concentrations, seasonal trends can be highly relevant, even for systems that do not add coagulants. Treatment modifications or other operational practices can also impact aluminum concentrations. Thus, water utilities should carefully monitor total aluminum concentrations, from the source through to the distribution system, as concentrations can change. System-specific monitoring plans should be developed to understand a number of issues (in no particular order): 1) whether residual aluminum concentrations increase or decrease from source to tap due to the use of any chemicals during treatment (e.g., for pH adjustment, coagulation, corrosion control, chloramination); 2) the fate and transport of aluminum in the distribution system and its impacts; and 3) for facilities using aluminum-based coagulants whether the coagulation process is optimized. The monitoring plan should capture all seasonal water quality conditions for comparison with the OG value of 0.100 mg/L. Samples should be collected at the entry point to the distribution system and at critical points within the distribution system; a sufficient number of sites should be sampled to be representative of the population served and the hydraulic zones within the distribution system. Areas with variable turbidity (e.g., particulate solids in the water) or conductivity (e.g., dissolved solids in the water) should be targeted. Turbidity and conductivity are general water quality parameters indicating changes in deposit accumulation or release in the distribution system. In the absence of turbidity and conductivity data, sites where indicator bacteria samples are collected can be used in the interim. Sample locations can be refined as water quality data is collected and trends are assessed. Monitoring the inlet and outlet of concrete reservoirs is also suggested to ensure that water quality is not aggressive to these facilities and thereby leaching aluminum into distributed water.
Total aluminum in drinking water, based on a locational running annual averageFootnote 1 of a minimum of quarterly samples taken in the distribution system, should be calculated for comparison with the MAC of 2.9 mg/L. However, as with all guidelines, any exceedance should be a signal to evaluate the situation, take corrective action(s) and consult with the responsible drinking water authority in the affected jurisdiction. The responsible authority may direct that a plan be developed and implemented to address the situation.
International considerations
Other national and international organizations have drinking water guidelines, standards and/or guidance values for aluminum in drinking water. Variations in these values can be attributed to the age of the assessments or to differing policies and approaches, including the choice of key study and the use of different consumption rates, body weights and source allocation factors.
The United States Environmental Protection Agency (US EPA), the European Union and Australia's National Health and Medical Research Council have not established health-based regulatory limits for aluminum in drinking water. Rather, these agencies and other international agencies have set guidance values ranging from 0.05 mg/L to 0.2 mg/L, based on aesthetic or operational considerations.
In its 2010 assessment of aluminum in drinking water, the World Health Organization (WHO) has calculated a non-regulatory health-based value of 0.9 mg/L but has highlighted the importance of not exceeding the practicable levels of 0.1-0.2 mg/L. The Canadian guideline value differs from the WHO's health-based value because Canada takes into consideration advancements in science since 2010. The WHO assessment is based on the Joint Food and Agriculture Organization of the United Nations/WHO Expert Committee on Food Additives' (JECFA) previous Provisional Tolerable Weekly Intake (PTWI) for aluminum of 1 mg/kg body weight per day (JECFA, 2007). JECFA has since revised their PTWI to 2 mg/kg body weight per day (JECFA, 2012) based on the key study, Poirier et al. (2011), that is also used to establish the Canadian guideline.
1. Exposure considerations
1.1 Sources and uses
Aluminum is the third most abundant metal in the Earth's crust. Mining and weathering of minerals result in the release of aluminum; consequently, it is found naturally in soils, groundwater, surface water and agricultural products such as vegetables, grains and meat. Canada is the world's third-largest producer of aluminum. The metal is used widely in construction materials (e.g., for buildings and infrastructure), vehicles, aircraft, electronics and packaging materials (NRCan, 2018). Aluminum compounds are also used by the pharmaceutical industry, in personal care products, in food packaging and as food additives. In addition, aluminum is used widely in treatment plants for drinking water, wastewater and industrial water. In drinking water treatment, aluminum salts are applied to remove turbidity, organic material and microorganisms. Statistics Canada (2013) reports that aluminum-based coagulants are used in the treatment process for 69.2% of surface waters and 6.7% of groundwater/groundwater under the direct influence of surface water. Aluminum has been found to leach from cement-based materials into drinking water (Leroy et al., 1996) and is also an impurity found in other chemicals used in water treatment (e.g., for pH adjustment) (NSF, 2018). In addition, aluminum can be added to drinking water as a result of using activated alumina to remove other contaminants (e.g., arsenic, fluoride).
1.2 Substance identity
Aluminum (Chemical Abstracts Service Registry No. 7429-90-5) is a ductile metal with a molecular weight of 26.98 and a vapour pressure of 1 mm Hg at 1 284 °C (ATSDR, 2008). The chemistry of aluminum in the aquatic environment is complex. The speciation, mobility and partitioning of aluminum are affected by numerous environmental characteristics, including the temperature, the presence/type of various ligands and the pH (ATSDR, 2008). Due to its reactive nature, dissolved aluminum does not exist in its elemental state but rather binds with either inorganic ligands (e.g., hydroxide, fluoride, sulphate) or organic ligands (e.g., natural organic matter [NOM]) to form numerous types of complexes. At low pH, the complex hydrated aluminum cation [Al(H2O)6]3+, also commonly known as "free aluminum" and abbreviated as Al3+, is the most soluble form of aluminum (Environment Canada and Health Canada, 2010). At high pH, the complex anion [Al(OH)4]-1 is most prevalent and is highly soluble. See Section 4.2 for further information on the effects of pH.
1.3 Exposure
As indicated in a Priority Substances List Assessment Report (Environment Canada and Health Canada, 2010), the main source for Canadians' exposure to aluminum is through food, followed sequentially by exposure through soil, drinking water and air (Table 1). Exposure through drinking water constitutes less than 5% of the average daily intake of aluminum. More specifically across the seven age categories used in the assessment, the percentage of total aluminum intake from drinking water ranges from 0 to 4.4%. Both extremes of this range relate to the age category of infants (0-6 months) and depend on whether infants are exclusively breastfed or not breastfed. The exposure for all other age categories ranges from 0.8% to 1.8%.
no data | Estimated mean daily intake of total aluminum (μg/kg bw per day) | ||||||
---|---|---|---|---|---|---|---|
Source of exposure | Infant (0-6 months) | Toddler (0.5-4 years) | Child (5-11 years) | Teen (12-19 years) | Adult (20-59 years) | Senior (>60 years) | |
Breastfed exclusively | Non-breastfed | ||||||
Drinking waterTable 1 footnote a | 0Table 1 footnote b | 11.84 | 5.01 | 3.94 | 2.24 | 2.35 | 2.47 |
Food and beverages | 12.2 | 85.0 | 268 | 341 | 270 | 143 | 113 |
Ambient air | 0.05 | 0.10 | 0.08 | 0.05 | 0.04 | 0.03 | |
Indoor air | 0.37 | 0.78 | 0.61 | 0.35 | 0.30 | 0.26 | |
Soils | 166 | 268 | 87 | 21 | 18 | 17 | |
TOTAL | 179 | 268 | 544 | 434 | 295 | 165 | 134 |
Note: Adapted from Environment Canada and Health Canada, 2010 |
Monitoring data submitted by the provinces and territories were analyzed to determine the median, mean, 90th percentile and maximum concentrations in municipal and non-municipal supplies; non-detectable results were included in the statistical analysis as half of the reported limit. Water monitoring data from the provinces and territories (municipal and non-municipal supplies; Table 2), the National Drinking Water Survey (Health Canada, 2017) (Appendix B) and Environment and Climate Change Canada (2017) (Appendix B) show that total aluminum:
- is detected in all water types but is variable across Canada;
- concentrations tend to be higher in surface water than in groundwater;
- concentrations are higher in rivers, likely due to high total particulate matter content;
- concentrations are generally low for raw, treated and distributed water, but the mean and 90th percentile levels of total aluminum in municipal surface water (treated and/or distributed) can exceed 0.100 mg/L;
- 90th percentile concentrations are lower in the distribution system than in the treated water for the three provinces that provided treated and distributed surface water data (i.e., Nova Scotia, Manitoba and Alberta), suggesting that aluminum may be precipitating in the distribution system;
- 90th percentile concentrations for non-municipal supplies (usually untreated groundwater) tends to be greater than the municipal raw groundwater concentration in the same jurisdiction; and
- maximum concentrations for non-municipal supplies (usually untreated groundwater) and municipal surface water (treated and/or distributed) can exceed the maximum acceptable concentration (MAC).
Jurisdiction (MDL mg/L) |
Water type (Non-municipal: ground/not specifiedTable 2 footnote a and municipal: ground/surface-raw, treated, distributedTable 2 footnote b) |
No. detects/ samples | Concentration (mg/L) | |||
---|---|---|---|---|---|---|
Median | Mean | 90th percentile | Max | |||
NewfoundlandTable 2 footnote 1 (0.005-0.01) |
Municipal: | |||||
Ground-raw | 42/102 | 0.005 | 0.021 | 0.030 | 0.280 | |
Ground-distribution | 629/1 686 | 0.005 | 0.019 | 0.030 | 1.000 | |
Surface-raw | 600/646 | 0.070 | 0.102 | 0.240 | 0.800 | |
Surface-distribution | 2 820/3 178 | 0.070 | 0.129 | 0.280 | 6.660 | |
Nova ScotiaTable 2 footnote 2 (0.005-0.010) |
Non-municipal: ground | 574/574 | 0.005 | 0.039 | 0.057 | 3.400 |
Municipal: | ||||||
Ground-raw | 77/133 | 0.006 | 0.007 | 0.012 | 0.032 | |
Ground-treated | 29/50 | 0.008 | 0.012 | 0.015 | 0.089 | |
Ground-distribution | 35/52 | 0.009 | 0.011 | 0.022 | 0.060 | |
Surface-raw | 88/88 | 0.082 | 0.101 | 0.212 | 0.501 | |
Surface-treated | 180/187 | 0.048 | 0.086 | 0.177 | 0.724 | |
Surface-distribution | 197/204 | 0.025 | 0.078 | 0.110 | 5.700 | |
New BrunswickTable 2 footnote 3 (0.001-0.025) | Non-municipal: ground | 90/443 | 0.013 | 0.014 | 0.013 | 0.580 |
Municipal: | ||||||
Ground-raw | 289/924 | 0.013 | 0.009 | 0.013 | 0.120 | |
Ground-distribution | 225/550 | 0.013 | 0.012 | 0.017 | 0.270 | |
Surface-raw | 104/139 | 0.029 | 0.039 | 0.085 | 0.228 | |
Surface-distribution | 338/391 | 0.018 | 0.027 | 0.052 | 0.300 | |
QuebecTable 2 footnote 4 (0.005-0.025) |
Municipal: | |||||
Ground-raw | 77/147 | 0.006 | 0.013 | 0.023 | 0.160 | |
Ground-treated | 1/2 | 0.020 | 0.020 | 0.034 | 0.037 | |
Ground-distribution | 32/67 | 0.005 | 0.010 | 0.015 | 0.110 | |
Surface-raw | 6/6 | 0.275 | 0.244 | 0.330 | 0.330 | |
Surface-treated | 6/6 | 0.029 | 0.084 | 0.200 | 0.360 | |
OntarioTable 2 footnote 5 (0.001) | Municipal: | |||||
Ground and surface-treated | 1 316/1 438 | 0.023 | 0.047 | 0.096 | 1.500 | |
Ground and surface-distributed | 1 212/1 387 | 0.024 | 0.042 | 0.109 | 1.340 | |
ManitobaTable 2 footnote 6 (0.0002-0.409) |
Non-municipal: ground | 51/144 | 0.002 | 0.010 | 0.014 | 0.266 |
Municipal: | ||||||
Ground-raw | 309/877 | 0.003 | 0.010 | 0.009 | 2.490 | |
Ground-treated | 194/606 | 0.003 | 0.008 | 0.007 | 0.381 | |
Ground-distribution | 72/96 | 0.002 | 0.007 | 0.006 | 0.392 | |
Surface-raw | 392/413 | 0.133 | 0.407 | 0.664 | 32.400 | |
Surface-treated | 396/443 | 0.035 | 0.169 | 0.330 | 7.970 | |
Surface-distribution | 71/72 | 0.022 | 0.150 | 0.284 | 3.900 | |
SaskatchewanTable 2 footnote 7 (0.0005-0.025/ 0.005-0.101 for non-municipal) |
Non-municipal: ground | 1 983/4 128 | 0.003 | 0.023 | 0.011 | 14.000 |
Municipal: | ||||||
Ground-raw | 187/216 | 0.001 | 0.010 | 0.008 | 0.740 | |
Ground and surface-treated | 288/293 | 0.011 | 0.106 | 0.272 | 2.030 | |
Ground and surface-distribution | 1 943/2 102 | 0.003 | 0.022 | 0.051 | 1.420 | |
Surface-raw | 147/148 | 0.040 | 0.139 | 0.203 | 3.173 | |
AlbertaTable 2 footnote 8 (0.003-0.020) |
Non-municipal: ground | 1 355/1 686 | 0.003 | 0.026 | 0.020 | 5.100 |
Municipal: | ||||||
Surface-raw | 147/148 | 0.180 | 0.626 | 1.732 | 6.200 | |
Surface-treated | 278/286 | 0.059 | 0.072 | 0.130 | 0.301 | |
Surface-distribution | 462/474 | 0.060 | 0.067 | 0.119 | 0.304 | |
British ColumbiaTable 2 footnote 9 (0.005-0.050) | Non-municipal: not specified | 313/352 | 0.020 | 0.059 | 0.050 | 3.000 |
YukonTable 2 footnote 10 (0.001-0.050) |
Municipal: | |||||
Ground-raw | 48/219 | 0.003 | 0.005 | 0.007 | 0.061 | |
Ground-treated | 11/68 | 0.005 | 0.005 | 0.005 | 0.025 | |
Surface-treated | 0/10 | 0.005 | 0.009 | 0.025 | 0.025 | |
CanadaTable 2 footnote c | Municipal: | |||||
Ground-treated | n/c | n/c | 0.008 | n/c | n/c | |
Ground-distribution | n/c | n/c | 0.015 | n/c | n/c | |
Surface-treated | n/c | n/c | 0.120 | n/c | n/c | |
Surface-distribution | n/c | n/c | 0.111 | n/c | n/c | |
Notes: n/c = not calculated MDL = method detection limit |
Aluminum is naturally present in many foods and certain aluminum-containing substances also have permitted uses as food additives. The highest concentrations of aluminum (>10 µg/g) among the composite food samples analyzed in the Canadian Total Diet Study between 2008 and 2012 were found in herbs and spices, baking powder, various baked goods, processed chicken products and chewing gum (Health Canada, 2016). The study's analysis of aluminum in infant formula found 0.040-0.171 µg/g in milk-based formula and 0.258-0.476 µg/g in soy-based formula (Health Canada, 2016). Comparable results were reported in the Canadian Food Inspection Agency's Children's Food Project and other targeted surveys (CFIA, 2018).
Canadians are also exposed to aluminum through consumer products (e.g., deodorants, creams, makeup and hair or nail products) and medications (e.g., anti-ulcer, anti-diarrheal, antiperspirants for hyperhidrosis). Notably, aluminum is present in antacids (~300-600 mg aluminum hydroxide per tablet) and represents an important source of exposure to individuals who consume antacids on a regular basis (ATSDR, 2008).
Aluminum concentrations in Canadian soil vary according to the sampling location, with average values ranging from 12 000 mg/kg in Nova Scotia to 87 633 mg/kg in British Columbia. The mean total aluminum concentration in Canadian soils, calculated from over 40 studies covering 10 provinces, was approximately 41 000 mg/kg (Environment Canada and Health Canada, 2010).
The levels of aluminum in ambient Canadian air also vary Concentrations range from the detection limit (~0.001 µg/ m3) up to 24.94 μg/m3 with a mean total aluminum concentration in particulate matter, 10 micrometres in diameter or less (PM10) of 0.17 µg/m3 (Environment Canada and Health Canada, 2010). Indoor air concentrations of aluminum are expected to be higher than outdoor air; however, they still do not constitute a significant exposure source (Environment Canada and Health Canada, 2010).
2. Health considerations
2.1 Kinetics
Absorption: Ingested aluminum is poorly absorbed through the gastrointestinal tract. While the acidic environment in the stomach favours the formation of the most soluble aluminum ion [Al(H2O)6]3+, the more neutral pH of the intestine results in the formation of insoluble aluminum hydroxide complexes, which are then generally excreted in feces. However, small amounts of aluminum that complexed with organic molecules in the stomach will still remain soluble at the higher pH of the small intestine. Absorption is generally greater with more soluble aluminum compounds; however, the absorption of aluminum through the stomach or intestines varies and depends heavily on the presence of chemical constituents from the diet and the types of complexes that aluminum forms with the dietary ligands (Zhou and Yokel, 2005). Intestinal absorption of aluminum may increase in the presence of anions, carboxylates (including citrate and lactate), fluoride and vitamin D supplements. Citrate (the conjugate base of citric acid) is one of the most important complexing agents relevant to aluminum uptake in humans. Blood and tissue levels of aluminum can be substantially increased through the intake of citric acid without further increasing the intake of aluminum itself. Conversely, the absorption of aluminum may decrease due to the presence of phosphates, silicones, polyphenols and folic acid supplements (ATSDR, 2008). The bioavailability of aluminum in drinking water has been measured in both human and animal studies. In humans, the absorption of aluminum complexed with citrate, chloride, hydroxide or lactate has been found to range between 0.01% and 0.65%. In experimental animals, the reported values range between 0.01% and 5.1% (Environment Canada and Health Canada, 2010). A likely estimate for aluminum bioavailability in both humans and animals is 0.3%, based on human studies by Stauber et al. (1999) and a critical review of animal data by Krewski et al. (2007).
Distribution: Aluminum primarily binds to transferrin; it is slowly taken up by tissues and organs and accumulates primarily in bone. To a lesser extent, aluminum can accumulate in the brain either by crossing the blood-brain barrier or through the choroid plexus in the cerebrospinal fluid of the cerebral ventricles. Aluminum is also detected in the lungs, skin, lower gastrointestinal tract, lymph nodes, adrenals, parathyroid glands and most soft tissue organs (EFSA, 2008; Environment Canada and Health Canada, 2010). Aluminum may also distribute to the placenta, fetus and breast milk (ATSDR, 2008). The distribution of aluminum may be influenced by other metals, including iron (negatively correlated with aluminum tissue accumulation), calcium and magnesium (deficiency may contribute to aluminum accumulation in the brain and bone) (EFSA, 2008).
Metabolism: The free form of aluminum (Al3+) binds easily to many substances. As a consequence, it is the affinity to the ligand and the metabolic fate of the complex that determines the metabolism of aluminum. Aluminum can form low-molecular-weight complexes with organic acids, amino acids, nucleotides, phosphates and carbohydrates that are quite stable. Aluminum can also form stable macromolecular complexes with proteins, polynucleotides and glycosaminoglycans. Some complexes are so stable that the aluminum cation cannot be exchanged for another cation. Because aluminum has a high affinity for these organic ligands, much of the aluminum in the body exists in the form of macromolecular complexes (ATSDR, 2008).
Elimination: In humans, upwards of 95% of the soluble aluminum that has been absorbed by the body is eliminated by the kidneys (Krewski et al., 2007). Individuals with compromised kidney function thus have an increased risk for aluminum toxicity (Willhite et al., 2014). The majority of the remaining portion is eliminated via biliary excretion in feces. To a much lesser extent, sweat, saliva and seminal fluid can also contribute to the elimination of aluminum (Krewski et al., 2007). The uptake and elimination of aluminum from the whole body were investigated in subjects injected with the radioisotope 26Al (Priest et al., 1995; Talbot et al., 1995; Priest, 2004). The majority of aluminum (~75%) was eliminated in urine within the first five days, however a follow-up study with one volunteer showed 4% of the injection remained after three years. The rate of elimination is influenced by a number of factors, including the presence of chemical complexes in the blood (e.g., aluminum citrate complexes are more readily eliminated than transferrin bound aluminum) (ATSDR, 2008). In addition, slower elimination and increased exposure with age contribute to the accumulation of aluminum in the body (NSCFS, 2013). In animal studies, elimination in rats was observed to occur more rapidly in well-perfused tissues (such as kidneys and lungs) than in poorly perfused tissues (such as bone and spleen), with half-lives of 2.3-113 days (Environment Canada and Health Canada, 2010). However, aluminum had a slower elimination rate from the brain, despite its being a well-perfused organ, with half-lives of 13-1 635 days (Krewski et al., 2007). Information on the allometric scaling of aluminum elimination rates was not available to extrapolate these results from rats to humans (Krewski et al., 2007).
Physiologically based pharmacokinetic modelling: No models applicable to the current risk assessment were identified.
2.2 Health effects
The database for the oral toxicity of aluminum is extensive, covering numerous endpoints (e.g., effects in bone, kidney, the nervous system and the immune system) and various types of exposure in both animals and humans (see Krewski et al. (2007), ATSDR (2008) and Willhite et al. (2014) for a more thorough review). The preponderance of the literature, however, focuses on neurotoxicity and reproductive/developmental toxicity; the emphasis on these endpoints is likely driven by findings in human case studies (i.e., encephalopathy in renal patients exposed to aluminum in dialysate and/or aluminum phosphate binders; cognitive impairment of preterm infants exposed to aluminum in parenteral nutritional solutions). An evaluation of the overall database clearly identifies the nervous system as the most sensitive target for aluminum toxicity (ATSDR, 2008). Other reviews also support this conclusion (EFSA, 2008; Environment Canada and Health Canada, 2010; JECFA, 2012). Consequently, studies examining neurological endpoints are the focus of the subsequent sections in this document. In addition, emphasis is placed on oral studies, as these are the most relevant for drinking water risk assessment. The previous review on aluminum by Environment Canada and Health Canada (2010) covers the literature up to 2008. All of the previous data is considered in the current assessment of aluminum in drinking water; however, the data presented herein focus on material published from 2009 to 2017.
2.3 Effects in humans
Despite aluminum's abundance in the environment, it is generally accepted that aluminum is not required by biological systems and does not participate in any essential biological processes (Exley, 2013). In terms of acute exposures, reports of short-lived nausea, vomiting, diarrhea, mouth ulcers, skin ulcers, skin rashes and arthritic pain were noted when up to 20 000 people were exposed to aluminum concentrations 500 - 3 000 times the World Health Organization (WHO) aesthetic value (0.2 mg/L) in an accidental contamination of water supplies in Camelford, United Kingdom (Lowermoor Incident Health Advisory Group, 1989). A number of follow-up studies to this acute exposure were conducted but did not demonstrate conclusive evidence of long-term effects (McMillan et al., 1993a, 1993b; Altmann et al., 1999; Exley, 2006; UK Committee on Toxicology, 2013).
Regarding longer-term exposures, a limited number of studies have investigated the effects of aluminum in healthy populations (see reviews in Krewski et al. (2007), ATSDR (2008) and Environment Canada and Health Canada (2010)). Several cross-sectional and ecological studies published after 2009 have investigated associations between aluminum and effects in bone (Dahl et al., 2014; Callan et al., 2015), kidney (Callan et al., 2015; Panhwar et al., 2016), reproduction and development (Huang et al., 2011; Giaccio et al., 2012; Karakis et al., 2014), body composition (Skalnaya et al., 2014; Cetin et al., 2017) and other endpoints in humans (Lindquist et al., 2011; Lv et al., 2011; Lind et al., 2012; Guo et al., 2013; Vandenplas et al., 2014).
The neurotoxicity of aluminum is well documented in human studies; however, many of these studies have been cases of medical treatment for specific disease conditions (e.g., patients with impaired kidney function). The association between exposure to aluminum and neurotoxicity endpoints in otherwise healthy individuals is less conclusive; such an association, specifically with Alzheimer's disease (AD), is the subject of much research and debate (Lidsky, 2014; Walton, 2014). Studies examining the link between aluminum and AD have focused on a number of areas, including the evaluation of aluminum concentrations in the body as it relates to AD. In the past, the results of these studies tended to be mixed (positive associations were noted in some studies but not in others) and this continues to be the finding of more recent investigations (Baum et al., 2010; Akatsu et al., 2011; Rusina et al., 2011; Bhattacharjee et al., 2013; Virk and Eslick, 2015; Mirza et al., 2017; Xu et al., 2018). Other studies that examined the concentration of aluminum in the brain in relation to the occurrence of beta-amyloid plaques and neurofibrillary tangles (primary features of AD pathogenesis) also noted mixed results (Strozyk et al., 2009; Walton, 2010; Exley et al., 2012).
Other recent studies examined the link between aluminum and the occurrence of various neurological diseases or disorders, other than AD, including amyotrophic lateral sclerosis (Garzillo et al., 2014), multiple sclerosis (Arain et al., 2015; Tamburo et al., 2015), attention deficit hyperactivity disorder (Nicolescu et al., 2010), autistic spectrum disorders (Albizzati et al., 2012), learning disabilities (do Nascimento et al., 2014) and cognitive dysfunction (Bakar et al., 2010). Most of these studies did not find a significant positive association between aluminum concentrations in the body and the respective neurological endpoint. Unfortunately, many of the studies were small in size, did not adjust for confounders and/or did not have adequate control populations.
A review outlined several epidemiological studies and investigated the association between exposure to aluminum in drinking water and the development of AD and other neurodegenerative disorders (JECFA, 2012). A large prospective study by Rondeau et al. (2009) found a significant association between high exposures to aluminum in drinking water (>0.1 mg/d) and the risks of cognitive decline, dementia and AD. However, the power of this study was low, with only 13 subjects (6 cases) having exposure ≥ 0.1 mg/day. In addition, there was a lack of information on exposure to aluminum through the diet, which was considered to account for 95% of the total oral exposure. A recent meta-analysis of cohort and case-control studies (including the study by Rondeau et al., 2009) found that chronic exposure to aluminum was associated with a 71% increased risk of AD (Wang et al., 2016). However, only eight studies were considered and half of these studies evaluated occupational exposures rather than drinking water exposures.
Overall, the epidemiological database provides only uncertain indications of an association between aluminum exposure and neurological diseases, including AD. Although recent reviews and international assessments consistently conclude that there is insufficient evidence for a causal link between exposure to aluminum and AD, there is also consensus that the hypothesis should not be dismissed (ATSDR, 2008; EFSA, 2008; Environment Canada and Health Canada, 2010; JECFA, 2012; Willhite et al., 2014). In addition to the absence of a clear point of departure needed for dose-response analysis, limitations in the epidemiological studies include a lack of individual exposure data, small sample sizes, poor disease ascertainment and failure to control for confounders. These limitations prevent the use of the study results in a quantitative risk assessment. However, the results of these studies can be used qualitatively to support the choice of the key endpoint used for quantitative assessment in animals.
2.4 Effects in animals
Exposure to aluminum is well known to result in a number of health effects in animal models. Reviews of these studies are found in Krewski et al. (2007), ATSDR (2008) and Willhite et al. (2014). Acute oral exposures of rats and mice to various aluminum compounds have resulted in median lethal dose (LD50) levels of 222 to 980 mg Al/kg (Ondreicka et al., 1966; Yokel and McNamara, 1985; Llobet et al., 1987; Vucetic-Arsic et al., 2013; Yu et al., 2016). Other studies, published after 2009, have noted effects in the bone at doses of aluminum chloride (AlCl3) >100 mg/L (Li et al., 2011a, 2011b, 2015; Sun et al., 2015, 2016, 2017), in the liver at doses of AlCl3 >34 mg/kg body weight (bw) per day (Turkez et al., 2010; Bhasin et al., 2012; Abdel Moneim et al., 2013; Belaid-Nouira et al., 2013b; Zhu et al., 2013; She et al., 2015; Ghorbel et al., 2016a) and in the kidneys at doses of AlCl3 >34 mg/kg bw per day (Abdel Moneim et al., 2013; Belaid-Nouira et al., 2013a; Wasana et al., 2015; Ghorbel et al., 2016b; Liu et al., 2016).
The vast majority of animal studies have investigated the potential for aluminum to cause neurotoxic, neurobehavioural and reproductive/developmental effects (including neurodevelopmental toxicity). Many of these experiments were designed to investigate the role of aluminum in the development of neurodegenerative diseases (including mechanistic studies), peripheral markers of aluminum neurotoxicity, or the protective properties of various agents against aluminum-induced toxicity. A summary of the most relevant studies published after 2009 is presented in Table 3. Included in this table are studies with exposure durations greater than 30 days in which aluminum was administered via drinking water (i.e., the most relevant exposure route). Studies conducted with gavage or dietary exposures were included only if they investigated multiple doses and could provide information about a dose-response relationship and point of departure that could be relevant in defining a health-based value. In Table 3, as well as throughout this document, where sufficient data was provided, the doses of the aluminum compound were expressed as mg aluminum to facilitate comparisons between studies. It should be noted that in many studies it was not clear whether the reported dose was reflective of the aluminum ion or of the aluminum compound (e.g., AlCl3·6H2O). Consequently, the dose as worded by the authors of the study is reported in the table unless otherwise indicated.
Neurotoxicity: The endpoints considered in the neurotoxicity studies listed in Table 3 include both histopathological effects (e.g., neuronal degeneration, vacuolization around the neuron, congestion in the blood vessels) and biochemical effects (e.g., oxidative stress responses, metal ion imbalances, altered neurotransmitter function). In these investigations, rats, mice and rabbits were exposed to aluminum for periods of 30 days to 18 months. The aluminum compounds investigated include aluminum chloride, aluminum sulphate and aluminum maltolate. Most of the studies investigated doses of aluminum that were significantly higher than human exposures would be under normal conditions. The lowest dose at which adverse neurotoxicity effects were observed was 10 mg/kg bw per day AlCl3 (≈2 mg Al/kg bw per day) (Rui and Yongjian, 2010). No data were found that addressed the reversibility of neurotoxic effects upon cessation of the exposure.
Neurobehavioural: The endpoints considered in the neurobehavioural studies include changes to reflexes, motor activity, learning, memory and sensory parameters. In these investigations, rats and mice were exposed to aluminum chloride for periods of 42 days to 14 months. Treatment with 100 mg/kg bw per day of aluminum chloride for 42 days (6 weeks) is a well-known model for inducing dementia (impaired spatial memory) in animals. The lowest dose at which adverse neurobehavioural effects were observed across the studies (i.e., lowest LOAEL [lowest-observed-adverse-effect level]) is 1.5 mg Al/kg bw per day, which was considered equivalent to human dietary aluminum exposure levels (Martinez et al., 2017a).
Reproductive/developmental toxicity (including neurodevelopmental toxicity): In the reproductive and developmental studies in Table 3, rats, mice and guinea pigs were exposed to various concentrations of aluminum chloride, aluminum citrate, aluminum sulphate and aluminum ammonium sulphate. These studies show that aluminum may affect reproductive parameters, including reproductive hormone levels, sperm counts, sperm motility, sperm morphology and testis histology. In addition, gestational and/or lactational exposure to aluminum can result in developmental effects that include decreased pup weight (often in the presence of maternal effects), delayed maturation, impaired neurobehaviour and changes to brain biochemistry. The lowest doses at which no adverse effects were observed are 8-14 mg Al/kg bw per day of aluminum sulphate (Hirata-Koizumi, 2011a) and 5-9 mg Al/kg bw per day of aluminum ammonium sulphate (Hirata-Koizumi, 2011b). These no-observed-adverse-effect levels (NOAELs) were based on decreased body weight gain and a slight but significant delay of the vaginal opening at the highest dose level in both studies. Interpretation of the study outcomes was confounded by treatment-related reductions in food and fluid consumption (likely due to the astringent taste and decreased palatability of the aluminum treated water). The authors stated that they could not separate the effects of the decreased water intake from the effects associated with aluminum treatment. Further, since other hormone-dependent events, such as those governing estrous cyclicity and post-natal anogenital distance, were not impacted in the aluminum-treated groups, the authors indicated that it was unlikely that aluminum had a clear impact on hormonal messaging during development. Therefore, if these results were disregarded, the next NOAEL dosage would be 30 mg Al/kg bw per day of aluminum citrate (Poirier et al., 2011). In addition, the LOAEL for reproductive effects is 1.5 mg Al/kg bw per day (Martinez et al., 2017b).
NOAEL/LOAEL (mg Al/ kg-day)Table 3 footnote a | Species, sex, number | Exposure duration | Compound and dose(s)Table 3 footnote b | Critical effect(s) | Key strength and/or weaknessTable 3 footnote c | Ref. |
---|---|---|---|---|---|---|
N/2 | Mice, ICR, (15/group) | 100 days | AlCl3; 0, 10, 50, 300 mg/kg bw per day via the diet | Neurotoxicity: Increased lipid peroxidation (MDA); decreased SOD; increased DNA damage (comet assay); increased mitochondrial DNA oxidative damage (8-OHdG) | Limited endpoints | (Rui and Yongjian, 2010) |
N/10 | Mice, Balb-c, M (10/group) | 5 weeks | AlCl3; 0, 50 mg/kg bw per day | Neurotoxicity: Increased lipid peroxidation (MDA); decreased antioxidant (GSH); decreased AChE and butyrylcholinesterase activity; activation of brain monoamine oxidase (MAO-A and MAO-B) but inhibition of cerebellar MAO-B | Single dose | (Linardaki et al., 2013) |
N/20 | Rats, Wistar, M (10/group) | 6 weeks | AlCl3; 0, 100 mg/kg bw per day | Neurotoxicity: Increased brain AchE; decreased acetylcholine, dopamine, noradrenaline, adrenaline and SOD; increased nitric oxide and H2O2, cortisol and adrenocorticotropic hormone; formation of amyloid plaques and necrosis of neurons | Single high dose | (ElBaz . et al., 2017) |
N/20 | Rats, Sprague-Dawley, M (6-8/group) | 8 weeks | AlCl3; 0, 100 mg/kg bw per day | Neurotoxicity: Decreased neurotransmitters, AchE; increased L-citrulline, nitric oxide and monoamine oxidase; increased tau, amyloid precursor protein, glial fibrillary acidic protein, ubiquitin, α-synuclein and Hsp 70; alterations in neurohistoarchitecture (loss of pyramidal and Purkinje cells) | Single high dose | (Singla and Dhawan, 2017) |
100/N | Mice, Tg2576 and Tg2576 /tau, F (6/group) | 4 or 10 months | AlCl3; 0, 100 mg/kg bw per day (as Al) | Neurotoxicity: Long-term Al intake did not accelerate the accumulation of Aβ in Tg2576 mice or accumulation of Aβ and tau in Tg2576/tau mice. | Single high dose | (Akiyama et al., 2011) |
N/101 | Rats, Wistar, M | 30 days | AlCl3; 0, 500 mg/kg bw per day | Neurotoxicity: Reduced catalase and GSH levels; mild degenerative changes in the prefrontal cortex; no evidence of amyloid deposits | Single high dose | (Akinola et al., 2015) |
N/? | Rats, Wistar, F (10/group) | 5 months | AlCl3; 0, 500 mg/kg bw per day i.g. for 1 month, then 1 600 ppm in drinking water for 4 months | Neurotoxicity: Increased lipid peroxidation in posterior brain; altered lipid metabolism | Single high dose | (Belaid-Nouira et al., 2012) |
N/? | Rats, Wistar, F (10/group) | 5 months | AlCl3; 0, 500 mg/kg bw per day i.g. for 1 month, then 1 600 ppm in drinking water for 4 months | Neurotoxicity: Reduced production of interleukin-6 (marker of inflammation) in the posterior brain; reduced immunoreactivity to GFAP (marker of astroglia activation) in the hippocampus and cerebral cortex; reduced number of GFAP-positive cells | Single high dose | (Belaid-Nouira et al., 2013c) |
N/? | Rats, Wistar, M (5/group) | 6, 12 or 18 months | AlCl3; 0.18, 0.72, 3.6 g/L | Neurotoxicity: Accumulation of aluminum in the brain varied by doses and exposure duration. Histopathological alterations in the dentate gyrus: destructive effect on subgranular layer and granular layer | No data on water consumption to calculate doses | (Hichem et al., 2014) |
N/35 | Rats, Sprague-Dawley, M (10/group) | 12 weeks | AlCl3; 0, 2 000 mg/L | Neurotoxicity: Increased Aβ in the hippocampus and cerebral cortex; histological evidence of shrunken and swollen neurons; reduced density of normal neurons | Single dose | (Zhang et al., 2013a) |
N/71 | Rats, Wistar (10/group) | 3 months | AlCl3; 0, 0.2%, 0.4%, 0.6% | Neurotoxicity: Decreased activities of protein kinase C and mitogen-activated protein kinase; reduced expression of extracellular signal-regulated kinases (ERK1/2) and Ca2+-calmodulin dependent protein kinase II (CaMKII) in hippocampus; attenuation of population spike amplitude of long-term potentiation (indicator of synaptic plasticity) from the hippocampal CA1 region | Actual daily Al doses not reported | (Wang et al., 2010) |
N/72 | Rats, Wistar (20/group) | 3 months | AlCl3; 0, 0.2%, 0.4% 0.6% | Neurotoxicity: Impact on Ras/ERK signal pathway: increased protein and mRNA expression of Ras; decreased expression of Raf1 and ERK2 in the hippocampi | Actual daily Al doses not reported | (Cui et al., 2012) |
N/? | Rats, Wistar, M (5/group) | 30 days | AlCl3; 0, 10, 100 ppm | Neurotoxicity: Oxidative stress induction (increased MDA, decreased SOD levels); activation of astroglia, microglia and infiltration of B-cells in the prefrontal cortex Some evidence of dose-response | Short-term study | (Akinrinade et al., 2015) |
N/N | Rabbits, New Zealand, M (5/group) | 10 weeks | Al sulphate; 0, 0.36 ppm (as Al) | Neurotoxicity: No increase in the number of beta-amyloid reactive neurons, but increased number of ABCA1-immunopositive neurons, in Al-treated rabbits fed a 2% cholesterol diet | Single dose | (Schreurs and Sparks, 2016) |
N/? | Mice, T 44 tau Tg and wild-type (5-13/ group) | 3, 6, 9, 12 months | Al maltolate; 0, 2 mM | Neurotoxicity: Accelerated tau aggregation, apoptosis and neurological dysfunction in mouse model with slow progressive tau accumulation | Single dose | (Oshima et al., 2013) |
N/1.5 | Rats, Wistar, M (6/group) | 60 days, 42 days | AlCl3·6H2O 1.5, 8.3, 100 mg/kg bw per day (as Al) | Neurobehaviour: Impaired recognition memory (object recognition memory test) | Well-conducted study | (Martinez et al., 2017a) |
N/10 | Mice, Balb-c, M (10/group) | 5 weeks | AlCl3; 0, 50 mg/kg bw per day | Neurobehaviour: Impaired long-term memory (passive avoidance task) | Single dose | (Linardaki et al., 2013) |
N/50 | Mice, Balb-c, M (5-9/group) | 42 days | AlCl3; 0, 250 mg/kg bw per day | Neurobehaviour: Deficits in learning and higher anxiety (fear extinction and open field tests) | Single high dose | (Farhat et al., 2017a) |
N/50 | Mice, Balb-c, M (5-9/group) | 42 days | AlCl3; 0, 250 mg/kg bw per day | Neurobehaviour: Impaired recognition memory (novel object recognition test), reduced sociability (social novelty preference test) | Single high dose | (Farhat et al., 2017b) |
N/71 | Rats, Wistar (10/group) | 3 months | AlCl3; 0, 0.2%, 0.4%, 0.6% | Neurobehaviour: Impaired memory (step-down test) | Actual daily Al doses not reported | (Wang et al., 2010) |
N/35 | Rats, Sprague-Dawley, M (10/group) | 12 weeks | AlCl3; 0, 2 000 mg/L | Neurobehaviour: Impaired spatial learning and memory (Morris water maze) | Single dose | (Zhang et al., 2013a) |
N/13 | Rats, Wistar, M (10/group) | 120 days | AlCl3; 0, 64.18, 128.36, 256.72 mg/kg bw per day | Reproductive: Suppression of testosterone and luteinizing hormone; decreased androgen receptor protein and mRNA expression | Did not account for Al in diet or for water consumed | (Sun et al., 2011) |
N/13 | Rats, Wistar, F (10/group) | 120 days | AlCl3; 0, 64.18, 128.36 and 256.72 mg/kg bw per day | Reproductive: Decreased levels of estrogen, progestogen, follicle-stimulating hormone and luteinizing hormone in serum | Did not account for Al in diet or for water consumed | (Wang N. et al., 2012) |
N/13 | Rats, Wistar, F (20/group) | 120 days | AlCl3; 0, 64, 128, 256 mg/kg bw per day | Reproductive: Damaged ovarian structure; altered iron, zinc and copper levels; decreased activities of Na(+)-K(+)-ATPase, Mg(2+)-ATPase and Ca(2+)-ATPase in the ovary; decreased follicle-stimulating hormone and luteinizing hormone protein expression | Did not account for Al in diet | (Fu et al., 2014) |
N/13 | Rats, Wistar, M (10) | 120 days | AlCl3; 0, 64.18, 128.36, 256.72 mg/kg bw per day | Reproductive: Decreased sperm count; increased sperm malformations; decreased testicular enzymes; altered iron, zinc and copper levels | Did not account for Al in diet or for water consumed | (Zhu et al., 2014) |
N/? | Rats, diabetic and non-diabetic, Wistar, M (10/group) | 30 days | AlCl3; 0, 250 ppm | Reproductive: Reduced sperm count and motility; decreased follicle-stimulating hormone; elevated estradiol levels | Single dose | (Akinola et al., 2016) |
N/? | Guinea pigs, M (13/group) | 13 weeks | AlCl3; 0, 300 mg/L | Reproductive: Decreased sperm count; increased sperm malformations; decreased testosterone; reduced gene and protein expression of StAR and P450scc | Single dose | (Dong et al., 2016) |
N/1.5 | Rats, Wistar, M (6/group) | 60 days, 42 days | AlCl3·6H2O; 1.5, 8.3, 100 mg/kg bw per day | Reproductive: Decreased sperm count, daily sperm production, sperm motility and normal morphological sperm; impaired testis histology; increased oxidative stress in reproductive organs; inflammation in testis | Well-conducted study | (Martinez et al., 2017b) |
N/? | Rats, Wistar, M (7-10/ group) | 6 months (3 generations) | Al sulphate; 0, 200, 400, 1 000 ppb | Reproductive: Lower testosterone levels; decreased sperm counts; higher percentages of immobile and abnormal sperm; decrease in testis weight; alterations in the histoarchitecture of the testes | Minimal study details reported | (Muselin et al., 2016) |
8-14/31-56 | Rats, Sprague- Dawley, M and F (24/sex/group) | 2 generations | Al sulphate; 0, 120, 600, 3 000 ppm | Reproductive/Developmental: No adverse effects on reproductive and fertility parameters; delay of the vaginal opening. | Confounding effects of decreased water intake | (Hirata-Koizumi et al., 2011a) |
5-9/36-61 | Rats, Sprague-Dawley, M and F (24/sex/group) | 2 generations | Al ammonium sulphate; 0, 50, 500, 5 000 ppm | Reproductive/Developmental: No adverse effects on reproductive and fertility parameters; delay of the vaginal opening | Confounding effects of decreased water intake | (Hirata-Koizumi et al., 2011b) |
N/N | Rats, Wistar, M and F (10/sex/group) | M: 28 days; F: 37-53 days | AlCl3; 0, 3.6, 18, 90 mg/kg bw per day (as Al); via gavage | Reproductive/Developmental: No reproductive, breeding or early post-natal developmental effects | GLP study | (Beekhuijzen, 2007, as reported in JECFA, 2012) |
30/100 | Rats, Sprague-Dawley, M and F (80/sex/group) | Gestation, lactation, until one year of age | Al citrate; 0, 30, 100, 300 mg/kg bw per day (as Al) | Developmental: Deficits in fore- and hind-limb grip strength and foot splay | GLP study | (Poirier et al., 2011) |
N/? | Rats, Wistar, M and F (5-10/ group) | Gestation, lactation, then direct exposure for 4 months | AlCl3; 0, 0.3% | Developmental: Reduced locomotor activity; increased anxiety; changes in the glial system; increased glial fibrillary acidic protein labelling and increased numbers of astrocytes in the brain; reduced locomotor activity; effects on dopaminergic neurons | Single dose | (Erazi et al., 2010; Erazi et al., 2011) |
N/60 | Mice, Swiss Webster, M and F | Gestation and lactation (PND 15) | AlCl3; 0, 300, 600 mg/kg bw per day | Developmental: Pre-weaning: dose-dependent decline in body weight gain and delays in eye opening and appearance of body hair fuzz; dose-dependent suppression of righting, rotating and cliff avoidance reflexes Post-weaning: dose-dependent deficits in locomotor activity and learning Various time points: dose-dependent decline in neurotransmitters in the forebrain |
No data on fluid consumption despite clear reduction of consumption | (Abu-Taweel et al., 2012) |
N/231 | Rats, Wistar, M and F (6-10/ group) | Lactation, then direct exposure for 3 months | AlCl3; 0, 0.2%, 0.4%, 0.6% | Developmental: Impaired spatial memory; changes to neuronal and synaptic ultrastructures in the hippocampus; electrophysiological impairment of late-phase long-term potentiation | Well-designed study, but comparatively high LOAEL | (Zhang et al., 2013b) |
N/231 | Rats, Wistar, M and F (6-10/ group) | Lactation, then direct exposure for 3 months | AlCl3; 0, 0.2%, 0.4%, 0.6% | Developmental: Long-term memory damage; changes to the neuronal and synaptic ultrastructure and repression of the cAMP-PKA-CREB signalling pathway | Well-designed study, but comparatively high LOAEL | (Zhang et al., 2014) |
N/10 | Rats, Wistar, F (6/group) | Gestation and lactation (PND 14) | AlCl3; 0, 50 mg/kg bw per day | Developmental: Decreased body weight, decreased enzymatic and non-enzymatic antioxidant levels, decreased AChE activities and increased levels of malondialdehyde and advanced oxidation protein product | Single dose | (Ghorbel et al., 2016c) |
N/? | Rats, Wistar, M and F (5/group) | Gestation lactation, then direct exposure for 4 months | AlCl3: 0, 3% | Developmental: Effects on serotonin neurotransmission in the brain; decreased RF glycoprotein (involved in the detoxification of cerebrospinal fluid) | Single dose | (Laabbar et al., 2014) |
Aβ: beta-amyloid; AChE: acetylcholinesterase; AlCl3: aluminum chloride; GLP: good laboratory practice; GSH: glutathione; i.g.: intragastric; MDA: malondialdehyde; PND:post-natal day; SOD: superoxide dismutase; |
Despite the wealth of research available on aluminum toxicity, many studies (including some of those listed in Table 3) have common limitations, such as the failure to quantify aluminum in the base diet of the study. Typical levels of 250 and 350 ppm aluminum in rodent chow could result in an additional 13-18 mg Al/kg bw per day in rats and 33-46 mg Al/kg bw per day in mice (Environment Canada and Health Canada, 2010). This becomes a significant issue when the test dose is actually less than the baseline dose in the diet but the combined dose has not been quantified or reported. Other study limitations include the use of single doses (which prevents the evaluation of a dose-response relationship and the determination of a point of departure), lack of detail on exposure conditions, failure to account for the acidity and decreased palatability of aluminum solutions in drinking water, exposure concentrations that are significantly greater than human exposures and short-duration exposures that are considerably shorter than the human lifespan.
2.5 Genotoxicity and carcinogenicity
Aluminum compounds are not generally considered to be gene mutagens but appear to act as clastogens and likely act through indirect mechanisms of action. As summarized in a study by the European Food Safety Authority (EFSA, 2008), the potential mechanisms of action include the cross-linking of DNA with chromosomal proteins, interactions with the microtubule assembly and mitotic spindle functioning, the induction of oxidative damage and the damaging of lysosomal membranes with the liberation of DNase. Despite producing DNA damage, the EFSA panel considered the genotoxicity of aluminum likely to be irrelevant for humans, given the presumed threshold and the low oral exposures. No conclusive evidence exists for the carcinogenicity of aluminum in animal models (Hackenberg, 1972; Schroeder and Mitchener, 1975a, 1975b; Oneda et al., 1994). The International Agency for Research on Cancer has not reviewed the carcinogenicity of aluminum.
2.6 Mode of action
Although numerous studies have attempted to elucidate the mode of action of aluminum toxicity, no single mechanism has been identified and it is likely that several processes are involved. The mechanisms implicated in aluminum neurotoxicity are summarized in several reviews (ATSDR, 2008; Environment Canada and Health Canada, 2010; Willhite et al., 2014) and include, among others, oxidative damage, inflammatory responses, changes in neuronal cytoskeletal proteins (neurofilament aggregates), altered cholinergic activity, effects on signal transduction pathways, membrane effects and metal ion imbalances. The literature to date provides no indication of a difference between the mode of action in animals and that in humans. A full analysis of the mode of action of aluminum toxicity was not conducted as it is not critical to the derivation of a health-based value (HBV) for aluminum.
2.7 Selected key study
Two key studies were considered in the risk assessment for aluminum: a neurodevelopmental study in rats by Poirier et al. (2011) and a reproductive (sperm quality) study in rats by Martinez et al. (2017b). In both of these studies, exposure to aluminum occurred via drinking water (the most relevant exposure route) and multiple doses of aluminum were used, allowing for the evaluation of the dose-response curve and identification of a point of departure. The study by Martinez et al. (2017b) had the lowest point of departure, while the study by Poirier et al. (2011) was a large, blinded study and was specifically designed to address previously identified research needs.
In the Poirier et al. (2011) study, the effects of aluminum citrate in rats were investigated in accordance with good laboratory practice (GLP) specifications and with a design based on Organization for Economic Co-operation and Development (OECD) Test Guideline 426: Developmental Neurotoxicity. Aluminum citrate was specifically selected as the most soluble and the most reliable aluminum compound able to cross into the blood and then cross the blood-brain barrier. In this double-blind study, male and female Sprague-Dawley rats were exposed to 30, 100 and 300 mg Al/kg bw per day in utero, through lactation and then in drinking water post-weaning until one year of age. Low concentrations (<10 µg/g) were present in the diet. There was significant morbidity and mortality in the male pups of the highest dose group and renal pathology associated with aluminum treatment was also observed in these male pups. In terms of reproductive outcomes, no effects on gestational length or on the number of offspring and litters were observed in the aluminum-treated groups as compared with controls. Delayed sexual development of both male and female pups was observed in the high-dose aluminum citrate group. This effect was considered treatment-related, but as body weights were also decreased, the authors were uncertain as to whether the developmental effects were secondary to decreases in body weight. In terms of neuromuscular development, the study noted a deficit in fore- and hind-limb grip strength and, to a lesser extent, in foot splay in the mid- and high-dose groups. For the present assessment, individual neuromuscular data were obtained and reviewed by Health Canada. A re-analysis of the data confirmed a significant deficit of the three neuromuscular endpoints in female rats, and a deficit in hind-limb grip strength for male rats. Since the aluminum citrate was delivered through the drinking water, the dose of aluminum varied with the extent of water consumption. In general, doses were at (or slightly under) target level at gestation, higher than target level during lactation and lower than target level during the remainder of the study. Although reduced water consumption affected aluminum uptake in the later stages of the study and may have confounded results, the effect on grip strength was still prominent in younger animals when exposure to aluminum was primarily due to in utero or lactational exposures when dams received appropriate or higher-than-target-level doses. Based on clinical observations, clinical biochemical changes and effects on renal pathology and neuromuscular function, the authors assigned a LOAEL of 100 mg Al/kg bw per day (target concentration) and a NOAEL of 30 mg Al/kg bw per day (target concentration).
In the Martinez et al. (2017b) study, the reproductive effects of low doses of aluminum chloride were investigated in male rats. In this non-OECD guideline study, rats were divided into two groups. The first group of rats was exposed for 60 days to 1.5 and 8.3 mg Al/kg bw per day as AlCl3‧6H20 via drinking water (equivalent to human dietary levels), whereas the second group was exposed for 42 days to 100 mg Al/kg bw per day as AlCl3‧6H20 via gavage (a known neurotoxicity model). In terms of reproductive effects, exposure to aluminum resulted in a decrease in: testis sperm count, daily sperm production in the testis and normal morphological sperm. Decreased sperm motility and impaired testis histology were also observed. No effects were observed on sperm number or transit time in the epididymis or cauda. Using the same study design, the authors also investigated the neurobehavioural effects associated with the same doses (Martinez et al., 2017a). No effects were observed on exploratory/locomotor activity (open field test), anxiety (elevated plus maze test) or pain threshold (hot plate test). However, exposure to both low aluminum doses, as well as the known neurotoxic dose, resulted in recognition memory impairment in the object recognition memory test. In the reproductive toxicity study, the effects seen at 8.3 mg Al/kg bw per day were occasionally greater and more significant than the effects observed at the neurotoxic dose (100 mg Al/kg bw per day). Although the authors state that further studies are necessary to explain these results, they suggest that the dose may not be the most important determinant of aluminum toxicity; rather, exposure conditions, intrinsic and individual characteristics, distribution and bioavailability through the body may also be important. In both the neurobehavioural and reproductive studies, increased markers of oxidative stress were noted in association with the effects and were highlighted as a potential mechanism of action.
Ultimately, the Poirier et al. (2011) study was chosen as the most appropriate study for the risk assessment. While the Martinez et al. (2017b) study was well conducted and had a lower point of departure, concerns were raised over the magnitude of the changes in sperm quality. The changes were often smaller at the 100 mg Al/kg bw per day dose than at the 8.3 mg Al/kg bw per day dose, despite the higher peak dose and cumulative exposure at the 100 mg Al/kg bw per day dose. No scientifically founded explanation could be offered for this unexpected finding. Furthermore, additional uncertainty factors would need to have been applied in the calculation of the HBV to account for the extrapolation from a LOAEL to a NOAEL and for a sub-chronic to a chronic study. This, in addition to the uncertainty factors for inter-species and intra-species variability, would have resulted in a level of uncertainty too high to derive a HBV. Confidence is placed in the study by Poirier et al. (2011) as it was a large blinded study specifically designed and undertaken to address previously identified research needs (JECFA, 2007). It evaluated multiple doses as well as multiple endpoints related to both developmental toxicity and neurotoxicity. In addition, the study was conducted according to GLP specifications and OECD guidelines. The selection of the Poirier et al. (2011) study is supported by JECFA (2012), which used the same key study and point of departure for its calculation of the provisional tolerable weekly intake of aluminum in the diet. The critical effect of decreased neuromuscular function, and specifically a decline in fore- and hind-limb grip strength, has been used in another international assessment (ATSDR, 2008) and is supported by previous research studies. Maternal exposure to aluminum during gestation and lactation has consistently resulted in decreases in grip strength in rodent pups (Donald et al., 1989; Golub et al., 1992a, 1995; Golub and Germann, 2001; Colomina et al., 2005). Studies examining adult exposures to aluminum have reported mixed results for grip strength (Donald et al., 1989; Golub et al., 1992a, 1992b, 1995, 2000; Oteiza et al., 1993; Drobyshev et al., 2018).
3. Derivation of the health-based value
Consistent with the Environment Canada and Health Canada (2010) report, the present assessment considers neurotoxicity and reproductive/developmental toxicity to be the critical endpoints of concern. These effects were observed across a variety of species (including humans), doses and exposure times, as well as through a variety of experimental assays. Since the release of the 2010 report, several robust key studies have been published, filling data gaps previously identified (JECFA, 2007; Environment Canada and Health Canada, 2010). As noted above, the study by Poirier et al. (2011) was selected as the basis for the current risk assessment. The NOAEL of 30 mg Al/kg bw per day identified by the authors is based on a dose-related decline in neuromuscular parameters (i.e., foot splay, fore- and hind-limb grip strength) as well as clinical observations, clinical biochemical changes and effects on renal pathology. Neuromuscular effects have been previously observed in both young and adult animals with similar points of departure, and it is unlikely that the young are more sensitive than adult populations (ATSDR, 2008).
Aluminum compounds in general have very low bioavailability (~0.3%) when ingested through drinking water. In the study by Poirier et al. (2011) aluminum citrate was used as it was considered to be the most soluble and most bioavailable (i.e., available for uptake from the digestive system) aluminum compound. A health-based value established from the point of departure with aluminum citrate is considered to be conservative and protective of exposure to all aluminum species because it assumes all of the aluminum is in the most bioavailable form. Potential modifying factors that could affect the bioavailability and consequent toxicity of aluminum in humans include the chemical form of aluminum, the presence of other chemical constituents from the diet and the types of complexes that aluminum forms with these dietary ligands. Depending on what is present in the gastrointestinal tract, the oral absorption of aluminum can vary at least 10-fold based on the type of complex alone (ATSDR, 2008; EFSA, 2008). For example, aluminum absorption may increase in the presence of carboxylic acids such as citrate (naturally present in many foods and fruit juices) but may decrease in the presence of silicone-containing compounds (likely due to the formation of insoluble hydroxyaluminosilicate).
Modelling of the dose-response data from the Poirier et al. (2011) study is considered inappropriate, given the low number of doses (for males: two doses plus the control due to significant mortality in the high dose group) and variability in the doses across the different study stages (the dosages received varied according to the animal's water consumption). Consequently, the data are not amenable to approaches such as benchmark dose modelling to calculate the point of departure. Therefore, the NOAEL of 30 mg/kg bw per day was retained as the point of departure.
Using the NOAEL of 30 mg/kg bw per day, the tolerable daily intake (TDI) for aluminum is calculated as follows:
Equation 1.
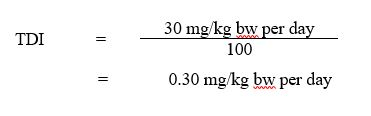
Equation 1 - Text Description
The TDI is 0.30 mg/kg bw per day. This is calculated by dividing 30 mg/kg bw per day by the uncertainty factor of 100.
where:
- 30 mg/kg bw per day is the NOAEL from Poirier et al. (2011), based on neuromuscular effects (i.e., a deficit in foot splay and fore- and hind-limb grip strength occurring at 100 mg/kg bw per day); and
- 100 is the uncertainty factor, selected to account for interspecies variation (×10), intraspecies variation (×10).
Using this TDI, the HBV for aluminum in drinking water is calculated as follows:
Equation 2.
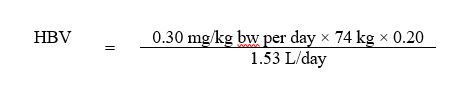
Equation 2 - Text Description
The HBV is 2.9 mg/L. This is calculated by multiplying 0.30 mg/kg bw per day by 74 kg, then by 0.20. This product is then divided by 1.53 L/day.
where:
- 0.30 mg/kg bw per day is the TDI derived above;
- 74 kg is the average body weight for an adult (Health Canada, in preparation);
- 0.20 is the allocation factor for drinking water. Given that food represents the main source of exposure, and drinking water was a minor contributor (0-4% for all age groups) to the total aluminum exposure, a floor value of 0.20 (20%) was applied. A floor value is used since the application of lower allocation factors results in unreasonably low guideline values which generally do not provide enhanced health protection because drinking water is only a small fraction of total exposure (i.e., the additional gain in terms of incremental protection is negligible) (Krishnan and Carrier, 2013);
- 1.53 L per day is the drinking water intake rate for a Canadian adult (Health Canada, in preparation). Due to its low volatility and low dermal absorption (Flarend et al., 2001; Pineau et al., 2012), exposure to aluminum from showering or bathing is unlikely to be significant. Consequently, a multi-route exposure assessment, as outlined by Krishnan and Carrier (2008), was not performed.
4. Analytical and treatment considerations
4.1 Analytical methods to detect aluminum
4.1.1 Standardized methods
Standardized methods available for the analysis of total aluminum in drinking water and their respective method detection limits (MDLs) are summarized in Table 4. MDLs are dependent on the sample matrix, instrumentation, and selected operating conditions and will vary between individual laboratories. Analyses for aluminum should be carried out as directed by the responsible drinking water authority in the affected jurisdiction. Water utilities should confirm that the method reporting limits are low enough to ensure accurate quantitation at concentrations below the MAC and the operational guidance (OG).
4.1.2 Online and portable colorimetric analyzers
Commercial online and portable analyzers are available for quantifying dissolved aluminum in source and drinking water, and analysis is generally based on standard method (SM) 3500-Al E (APHA et al., 1995) or SM 3500-Al B (APHA et al., 2017). Acidification of the sample prior to analysis is needed for the measurement of total aluminum. Some online analyzers have an internal digestion unit to measure both dissolved and total aluminum. These analyzers can be used to obtain a rapid or continuous (online units only) indication of changes to aluminum concentrations, which are critical for process monitoring within a water treatment plant (Haught and Fabris, 2002).
In general, commercial online methods are capable of measuring aluminum concentrations between 5 µg/L and 1 500 µg/L, with higher concentrations requiring dilution. The detection limits range from 1 µg/L to 10 µg/L. To accurately measure aluminum using these units, water utilities should develop a quality assurance and quality control (QA/QC) program such as those outlined in SM 3020 (APHA et al., 2017). In addition, periodic verification of results using an accredited laboratory is recommended. Water utilities should check with the responsible drinking water authority in the affected jurisdiction to determine whether results from these analyzers can be used for compliance reporting.
Method (Reference) | Methodology | MDL (µg/L) | Interferences/Comments |
---|---|---|---|
United States Environmental Protection Agency (US EPA) Methods | |||
EPA 200.5 Rev. 4.2 (US EPA, 2003) |
Axially viewed inductively coupled plasma-atomic emission spectrometry | 2.2 | Matrix interferences: calcium, magnesium and sodium >125 mg/L and silica >250 mg/L |
EPA 200.7 Rev. 4.4 (US EPA, 1994a) |
Inductively coupled plasma-atomic emission spectrometry | 20 | Matrix interferences: total dissolved solids >0.2% weight per volume |
EPA 200.8 Rev. 5.4 (US EPA, 1994b) |
Inductively coupled plasma-mass spectrometry | 1Table 4 footnote aa-1.7Table 4 footnote b | Matrix interference: total dissolved solids >0.2% weight per volume |
EPA 200.9 Rev 2.2 (US EPA, 1994c) |
Graphite furnace atomic absorption spectrometry | 7.8 | Use of hydrochloric acid may cause chloride ion vapour state interferences. Elevated aluminum in palladium matrix will cause elevated blank absorbances. |
American Public Health Association (APHA) Standard Methods (APHA et al., 2017, except where noted) | |||
SM 3111D and SM 3111E |
Direct (SM 3111D) or extraction (3111E) nitrous oxide-acetylene flame atomic absorption spectrometry | 100 | SM 3111E: Applicable for determination of aluminum concentrations <900 µg/L; matrix interference: iron >10 mg/L |
SM 3113B | Electrothermal atomic absorption spectrometry | 3 | Not specified |
SM 3120B | Inductively coupled plasma-mass atomic emission spectrometry | 40 | Matrix interference: total dissolved solids >1 500 mg/L |
SM 3125 | Inductively coupled plasma mass spectrometry | 0.03 | Matrix interference: total dissolved solids >0.5% weight per volume |
SM 3500-Al B (Portable analyzers) |
Colorimetric method using eriochrome cyanine R dye and spectrophotometer (535 nm) | 6 | Fluoride and phosphates may cause interferences. Procedures and correction factors may be needed to obtain accurate measurements. |
SM 3500-Al E (Online analyzers) (APHA et al., 1995) |
Colorimetric method using pyrocathechol violet and spectrophotometer (580 nm) | 7-10 | Not specified |
4.1.3 Sample preservation and preparation
Total aluminum includes both the dissolved and particulate (suspended) fractions of aluminum in a water sample and is analyzed using methods for total recoverable aluminum. Analysis of total aluminum is needed for comparison to the MAC and OG. Determining the concentration of both the dissolved and particulate fractions may be necessary when conducting bench- or pilot-scale testing or for process monitoring (see Section 4.2.1.1).
Sample processing considerations for analysis of aluminum in drinking water can be found in the references listed in Table 4. Accurate quantification of dissolved, particulate and total aluminum in samples is dependent on the proper sample preservation and processing steps. SM 3030B provides guidance on filtration and preservation (acidification) procedures for the determination of dissolved or particulate metals (APHA et al., 2017). It is important to note that in order to determine dissolved aluminum concentrations, samples should be filtered and the filtrate acidified to pH <2 at the time of collection (not at the laboratory). If this is not possible, the sample should be collected in an unpreserved bottle, cooled to 4 °C and delivered to the laboratory without delay for filtering and acidifying (APHA et al., 2017). Delineation between dissolved and particulate fractions in a sample is dependent on the filter type and pore size; therefore, water utilities that may have smaller particles or colloids present in the water should consider whether the standard filter size (0.4-0.45 µm pore-diameter membrane) will be suitable.
US EPA methods 200.7 and 200.8 and SM 3111D, SM 3113B, SM 3120B do not require hot acid digestion for total recoverable metals unless the turbidity of the sample is greater than 1 nephelometric turbidity unit (NTU). APHA et al. (2017) recommends verifying whether adequate recovery of metals has occurred in different sample matrices by comparing digested and undigested samples. Microwave-assisted digestion (SM 3030K) is recommended for analysis of total recoverable metals using standard methods that are based on inductively coupled plasma-mass spectrometry.
4.2 Treatment considerations
The form of aluminum (e.g., particulate or dissolved) that will be present depends on a wide variety of environmental parameters, including pH, temperature, NOM and the presence of inorganic ligands such as fluoride, sulphate, silicate and phosphorus (Environment Canada and Health Canada, 2010). Aluminum is highly insoluble in the near neutral pH range (Appelo and Postma, 1996). Depending on water quality conditions various chemical precipitates may form, involving oxide, hydroxide, silicate or phosphate (Snoeyink et al., 2003; Friedman et al., 2010). In low pH or high pH conditions, most forms of aluminum become highly soluble.
Aluminum solubility is also influenced by temperature. For aluminum sulphate (alum), the pH of minimum solubility occurs at 6.2 at 20 °C and shifts to 6.7 at 5 °C (see Figure 1). At the pH of minimum solubility, soluble aluminum concentrations of 0.005-0.014 mg/L are expected (-6.7 M and -6.3 M, respectively, in Figure 1). This increases dramatically to 27 mg/L at pH 9.7 and 20 °C (-3 M) (Van Benschoten et al., 1992).
For pre-hydrolyzed forms of aluminum (e.g., polyaluminum chloride; PACl), the pH of minimum solubility for a coagulant with high basicity occurs at 6.4 at 20 °C and shifts to 6.9 at 5 °C (see Figure 2). As a result, PACl coagulants can generally be used at higher pH values (Pernitsky, 2003) and over a wider temperature range at lower coagulant doses (Matilainen et al., 2010). However, at pH values less than the pH of minimum solubility, dissolved aluminum concentrations increase much more steeply than for alum (Pernitsky and Edzwald, 2006). Water utilities that use medium basicity PACl, aluminum chlorohydrate, polyaluminum sulphate or polyaluminum silica sulphate coagulants should consult the solubility curves of Pernitsky and Edzwald (2003) regarding the effect of pH. Pre-hydrolyzed forms of aluminum (e.g., polyaluminum coagulants) are pre-neutralized and therefore less acidic. The basicity, which is normally given, provides an indication of how "acid-neutralized" the coagulant is. For example, a product with 50% basicity is 50% pre-neutralized relative to alum. This can be important when considering post-coagulation pH adjustment to maintain water quality in the distribution system (see Sections 4.3 and 5).
The solubility curves shown in Figures 1 and 2 were developed from chemical thermodynamics and verified by the experimental data of Pernitsky and Edzwald (2003, 2006) and VanBenschoten and Edzwald (1990a). The figures show the residual aluminum concentration in equilibrium with the amorphous precipitate Al(OH)3 in coagulation. Conditions above the curve indicate oversaturation and those below the curve indicate subsaturation.
Aluminum solubility in natural waters does not necessarily behave in the same way as predicted in Figures 1 and 2; aluminum concentrations lower than predicted are reported (Kimura et al., 2013). Thus, solubility curves like those shown in Figures 1 and 2 have been developed for natural waters to demonstrate that optimum coagulation (for turbidity, NOM and residual aluminum concentration) occurs within the pH range of minimum solubility (Driscoll and Letterman, 1988, 1995; Jekel and Heinzmann, 1989; Halton, 2001; AWWA, 2011a; Health Canada, 2018a). Optimum pH ranges, as reported in the body of literature, are summarized in Table 5.
Coagulant | Cold water (<10 °C) | Warm water (>10 °C) |
---|---|---|
Alum | 6.5 to 7 | 6.0 to 6.5 |
PACl | 6.8 to 7.3 | 6.3 to 6.8 |
Thus, pH and temperature will have an important influence on the aluminum concentration in treated water and potentially on aluminum deposition and accumulation within distribution systems.
Figure 1. Alum solubility curves based on theory and experimental data presented in Pernitsky and Edzwald (2003, 2006)
(Adapted from JWSRT - AQUA Volume 55, Issue 2, pp. 121-141, with permission from the copyright holders, IWA Publishing)
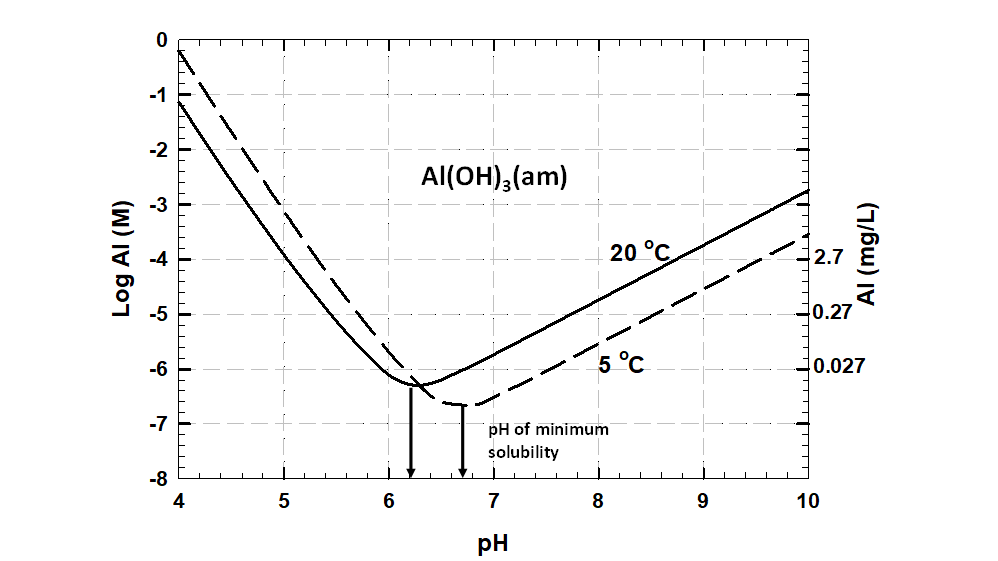
Figure 1 - Text Description
A graph that shows the solubility for alum. The left vertical axis shows the aluminum concentration on a log scale in moles ranging from -8 to 0 and the right vertical axis shows the aluminum concentration in mg/L. The horizontal axis shows pH ranging from 4 to 10. The solubility curve is shaped like a parabola with the point of minimum solubility at the base. The left side of the parabola raises at a slope of 2.5 while the right side raises at a slope of 2. Two solubility curves are provided – one for 20 °C with the base at pH 6.2 and one for 5 °C shifted to the right with the base at pH 6.7.
Figure 2. PACl solubility curves based on theory and experimental data presented in Pernitsky and Edzwald (2003, 2006)
(Adapted from JWSRT - AQUA Volume 55, Issue 2, pp. 121-141, with permission from the copyright holders, IWA Publishing).
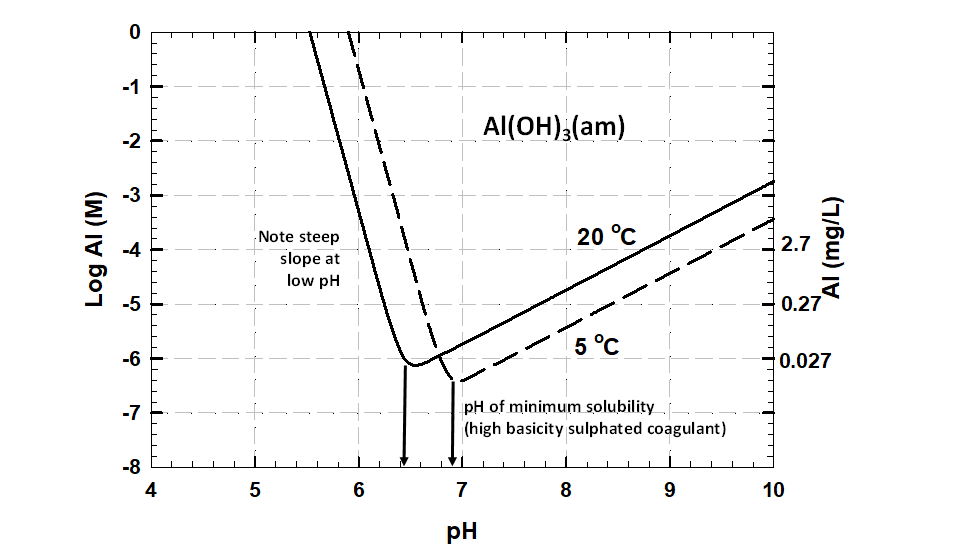
Figure 2 - Text Description
A graph that shows the solubility for polyaluminum chloride. The left vertical axis shows the aluminum concentration on a log scale in moles ranging from -8 to 0 and the right vertical axis shows the aluminum concentration in mg/L. The horizontal axis shows pH ranging from 4 to 10. The solubility curve is shaped like a parabola with the point of minimum solubility at the base. The left side of the parabola increases sharply at a slope of 7 while the right side increases more gradually at a slope of 3. Two solubility curves are provided – one for 20 °C with the base at pH 6.4 and one for 5 °C shifted to the right with the base at pH.
It is important to minimize the aluminum concentration in treated water because it can impact water quality:
- aluminum concentrations exceeding the MAC have been reported. Kim et al. (2011) measured dissolved aluminum concentrations in the order of 2-7 mg/L between pH 9 and 10 in batch dissolution tests of corrosion products from a lead pipe. Cantwell et al. (2012) reported total aluminum concentrations of 1 060-4 610 μg/L between pH 7.1 and 7.6 during an eight-month pipe loop study using lead service lines harvested from the distribution system. Locco et al. (2018) reported total aluminum concentrations ranging from 0.423- 5.85 mg/L and 1.64-38.2 mg/L when pilot testing unidirectional flushing velocities of 0.7 m/s and 1.3 m/s, respectively. These studies highlight that aluminum can accumulate in the distribution system and be released under certain conditions. Table 2 confirms that drinking water concentrations above the MAC occur. Although sampling conditions were not provided by the provinces and territories, the maximum values reported for municipal surface water (treated or distribution) and non-municipal (typically untreated groundwater) were above the MAC in some jurisdictions.
- aluminum precipitates can trap and protect microorganisms, potentially impairing the efficacy of disinfection processes at the treatment plant and within the distribution system (Letterman and Driscoll, 1988);
- aluminum precipitates in the distribution system can influence the concentration of lead and copper (Kvech and Edwards, 2001), adsorb and release arsenic and chromium (Kim et al., 2011) and act as an accumulation sink for other contaminants (Snoeyink et al., 2003; Friedman et al., 2010);
- precipitates of aluminum can adsorb or co-precipitate lead and copper, contributing to co-accumulation and the risk of particulate lead/copper releases (Cantwell et al., 2012; Knowles et al., 2015; Cantor, 2017);
- aluminum hydroxide has a strong affinity for manganese at pH >7.5 (Wang et al., 2012a);
- aluminum can interfere with lead and copper corrosion control strategies involving orthophosphate passivation by preventing the formation of protective scales (AWWA, 2011b; Wasserstrom et al., 2017).
The precipitation of aluminum in the distribution system can also result in operational issues:
- decreased carrying capacity of watermains and associated pressure loss or increased pumping costs (Baylis, 1953; Hudson, 1966; Cooper and Knowles, 1975; Foley, 1980; Costello, 1984; Kriewall et al., 1996; Grigg, 2010); even a thin layer of aluminum deposits can significantly reduce transmission capacity (Hudson,1966);
- aluminum deposition on water meters, causing them to malfunction and on service lines causing low household water pressure (Halton, 2001);
- the appearance of turbid or discoloured water (e.g., "milk-coloured" or "cloudy" water) (Costello, 1984; NHMRC and NRMCC, 2011; Dietrich, 2015; Locco et al., 2018).
At high concentrations (5-6 mg/L) aluminum may cause an unpleasant taste, and at very high concentrations (100-500 mg/L) the water may feel "sticky" (Hrudey and Hrudey, 2014). High and very high concentrations have occurred as a result of accidents at full-scale water treatment plants using aluminum-based coagulants (Lowermoor Incident Health Advisory Group, 1989; DWQR, 2011; UK Committee on Toxicology, 2013). This highlights the need for standard operating procedures, alarms and interlocks, and contingency plans when dealing with water treatment chemicals.
4.2.1 Municipal-scale treatment
For naturally occurring aluminum in source water, the only known effective treatment technology is coagulation. This is a complex treatment technology that is typically not used for small systems or groundwater supplies. In cases where aluminum removal is required and coagulation is not feasible, the responsible drinking water authority in the affected jurisdiction should be contacted to discuss possible options.
When coagulation is used, the choice of coagulant will depend on the characteristics of the water to be treated (e.g., pH, alkalinity), corrosion potential (e.g., changes to the chloride:sulphate mass ratio), secondary impacts related to the handling of process residuals (e.g., backwash water) and economic feasibility. Available coagulant choices (e.g., aluminum- and iron-based coagulants, organic polyelectrolytes, composite coagulants and novel coagulants) are discussed elsewhere (AWWA, 2011a; MWH, 2012; Sillanpää and Matilainen, 2015; Sillanpää et al., 2018).
Coagulation has multiple objectives, and optimum coagulation conditions necessitate a coagulant dose and a pH that:
- maximize the removal of turbidity (particles) by downstream processes;
- maximize the removal of NOM (disinfection by-product precursors); and
- minimize the coagulant residual in treated water.
Thus, coagulation should be viewed as an "integrated" process that considers turbidity, NOM and the coagulant residual for the full range of water quality conditions (Eikebrokk, 1996). It is important to note that for many water supplies, coagulant dosing is controlled or influenced by NOM rather than turbidity (e.g., particles). For the pH conditions of most water sources (pH 6-8), NOM and particles carry a negative charge that becomes more negative with increasing pH. However, the negative charge of NOM is typically between 5 and 15 µeq/mg carbon, while that of particles is between 0.05-0.5 µeq/mg particle, depending on the particle type (Edzwald, 1993). Pernitsky and Edzwald (2006) estimated the charge for both the NOM and particle components for a variety of typical North American water sources. The authors reported that, in most cases, the particle charge was <1 µeq/L whereas the NOM charge was 7 to 50 times greater depending on the concentration and character of NOM. Table 6 compares the coagulant dose required to neutralize the negative charge of typical water sources. Additional guidance regarding NOM is provided in Section 4.2.1.1 of this document and in the Guidance on natural organic matter in drinking water (Health Canada, 2020a).
Water | Source type | Turbidity (NTU) |
Total organic carbonTable 6 footnote b (mg/L) |
SUVATable 6 footnote c (L/mg∙m) |
Dose to neutralize charge (mg/L as Al) |
---|---|---|---|---|---|
1 | Turbid, low organic carbon river | 16 | 2.5 | 2.2 | 0.8 |
2 | Low turbidity, low organic carbon reservoir | 0.8 | 2.8 | 3.0 | 1.5 |
3 | High colour reservoir | 0.7 | 6.1 | 4.5 | 4.2 |
Source-specific treatability studies, including bench- and/or pilot-scale testing, are essential to select effective strategies for the full range of water quality conditions (Valade et al., 2009; Huck and Sozański, 2011). While some coagulants provide a wider operational window with respect to pH, it is noteworthy that for all metal coagulants the pH of minimum solubility increases as temperature decreases (Pernitsky, 2003). Thus, treatability studies should be conducted for both cold (<10 °C) and warm (>10 °C) water conditions. Coagulant selection can be particularly important during cold water conditions when rates of coagulation are slower (Driscoll and Letterman, 1988; Pernitsky and Edzwald, 2006; AWWA, 2011c). Water utilities may require seasonal changes (e.g., pH adjustment) to manage the impacts of temperature and effectively treat water throughout the year. The lack of a source-specific treatability study may result in the selection of inappropriate treatment, an increase in corrosion potential or other unintended consequences.
4.2.1.1 Use of aluminum-based coagulants
When aluminum-based coagulants are added to the water, chemical reactions occur with particles, as well as with the organic matter naturally present in the source water. The organic matter acts as a ligand that complexes the positively charged aluminum ions, exerting a coagulant demand that must be overcome before flocculation can occur (Edzwald and Haarhoff, 2012). If the coagulant dose is insufficient to overcome this demand, aluminum-NOM complexes form and remain in dissolved or colloidal form. These complexes are not incorporated into filterable precipitates and thus, pass through the filter resulting in elevated aluminum residuals in treated water and suboptimal particle removal (Jekel and Heinzmann, 1989; Edzwald and Van Benschoten, 1990; Van Benschoten and Edzwald, 1990a; Srinivasan et al., 1999; Edzwald and Kaminski, 2009). The coagulant dose must be increased to allow the dissolved/colloidal aluminum-NOM complexes to form filterable flocs (Srinivasan et al., 1999; Halton, 2001; Pivokonsky et al., 2006; Yan et al., 2008). Alternatively, a coagulant aid can be added (Logsdon et al., 2002; Pernitsky, 2019). It is important to note that some NOM fractions cannot be removed by coagulation at any pH or dose. Other treatment options for these fractions are discussed in the Guidance on natural organic matter in drinking water (Health Canada, 2020a).
Under acidic conditions, over-dosing can also increase the aluminum residual (Van Benschoten and Edzwald, 1990a). Post-treatment precipitation of particles causing turbidity, as well as deposition and accumulation within distribution systems, can occur with changes in pH and temperature (Snoeyink et al., 2003; Pernitsky and Edzwald, 2006).
A review of paired raw and treated water samples for surface water treatment plants in three provinces (Nova Scotia, Manitoba, Alberta) found a decrease in aluminum concentrations for 70-82% of the samples. Increased concentrations in treated water tended to occur when raw water aluminum concentrations were low (see Table 7). The concentration increases clearly highlight the impacts of improper coagulation on aluminum residuals and the potential for treated water concentrations to exceed the MAC, although the use of other water treatment chemicals may have contributed to the increase (see Section 4.2.1.3). The decreased concentrations provide an indication of the low concentrations (0.010-0.032 mg/L) that can be achieved.
Jurisdiction | Number of paired samples | Increased concentrations | Decreased concentrations | ||
---|---|---|---|---|---|
Nova ScotiaTable 7 footnote 1 | 54 samples from 24 facilities | n | 15/54 (28%) | n | 39/54 (72%) |
Raw | 0.071 mg/L | Raw | 0.180 mg/L | ||
Treated | 0.724 mg/L | Treated | 0.010 mg/L | ||
% increase | 920% | % decrease | 94.4% | ||
ManitobaTable 7 footnote 2 | 154 samples from 34 facilities | n | 46/154 (30%) | n | 108/154 (70%) |
Raw | 0.047 mg/L | Raw | 32.4 mg/L | ||
Treated | 7.97 mg/L | Treated | 0.032 mg/L | ||
% increase | 16 714% | % decrease | 99.9% | ||
AlbertaTable 7 footnote 3 | 136 samples from 3 facilities | n | 24/136 (18%) | n | 112/136 (82%) |
Raw | 0.052 mg/L | Raw | 5.68 mg/L | ||
Treated | 0.256 mg/L | Treated | 0.025 mg/L | ||
% increase | 392% | % decrease | 99.6% | ||
A review of 10 full-scale case studies assessed the achievable aluminum residual concentration for the range of temperatures experienced in Canada (Health Canada, 2018a). Findings are summarized in Table 8 and show that water treatment plants adding aluminum-based coagulants can exceed 0.100 mg/L at some time, during either cold or warm water conditions, when pH is not seasonally adjusted. Plants coagulating at acidic pH tend to experience higher aluminum concentrations in cold water conditions, because this is when they are operating furthest from the point of minimum solubility (see Figures 1 and 2). Conversely, plants coagulating at alkaline pH generally experience higher aluminum concentrations in warm water conditions, because this is when they are operating furthest from the point of minimum solubility. Other published literature documented similar findings (Van Benschoten and Edzwald, 1990b; Anderson et al., 1998; Halton, 2001; Kundert et al., 2004). In addition, water treatment plants with changes in NOM content experienced elevated aluminum residual concentrations due to inadequate coagulant dose. Increasing the coagulant dose decreased aluminum residual concentrations from 0.16-0.50 mg/L to 0.06-0.07 mg/L (Srinivasan et al., 1999; Anderson et al., 2017; Health Canada, 2018a).
pH conditions | Water temperature at which high aluminum residuals occur | Aluminum concentrations |
---|---|---|
Constant acidic pH (i.e., no seasonal adjustment) | ≤5 °C | Increase from <0.05 mg/L to ~0.300 mg/L |
pH seasonally adjusted | Not applicable | Below 0.06 mg/L for all seasonsTable 8 footnote b except for two measurementsTable 8 footnote c |
Constant alkaline pH (i.e., no seasonal adjustment) | ≥15 °C | Increase from <0.05 mg/L to ~0.400 mg/L |
Strict pH control and adequate coagulant dosing are necessary to minimize aluminum residual concentrations in treated water (Driscoll and Letterman, 1995). Monitoring streaming current or zeta potential can help determine if optimal coagulation conditions exist (Morfesis et al., 2009; AWWA, 2011c; Kundert et al., 2014; McVicar et al., 2015; Pernitsky, 2019). Maximum turbidity and NOM removals have been reported when the coagulant particle charge is near neutral (Sharp et al., 2006; Sharp, 2015). Inefficient filtration can also result in higher residual aluminum concentrations (Driscoll and Letterman, 1988). Thus, when optimizing coagulation, it is recommended that a filter effluent turbidity goal of 0.1 NTU be established to minimize aluminum residuals (Jekel and Heinzmann, 1989; Van Benschoten et al., 1992).
Jar testing is a helpful tool to optimize the coagulation process and test alternate coagulants and/or flocculant aids (AWWA, 2011c). When conducting jar tests, the integrated goals of coagulation—turbidity (particles), NOM (disinfection by-product precursors) and coagulant residual—should be considered. Surrogates for measuring NOM include, but are not limited to, total or dissolved organic carbon, ultraviolet absorbance at 254 nm (UV254) and DBP formation potential (Health Canada, 2020a). Total and dissolved aluminum should be measured because turbidity measurements do not provide information related to soluble aluminum.
It is possible that flocs will not settle during jar testing as studies indicate that low-density NOM flocs are more amenable to flotation than to sedimentation (Plummer et al., 1995; Edzwald and Kelley, 1998; Edzwald et al., 1999, 2000, 2003; Harrington et al., 2001; Edzwald, 2010; Gregory and Edzwald, 2011). Thus, it may be necessary to use a jar test procedure that includes a clarification process such as dissolved air flotation (AWWA, 2011c) or ballast media (Lapointe and Barbeau, 2019).
Process monitoring of residual aluminum should include total and dissolved aluminum concentrations. Dissolved aluminum provides an indication of the suitability of the coagulation pH, while particulate aluminum indicates the performance of filter operations. In this case and for bench- or pilot-scale testing, it is acceptable to consider particulate aluminum to be the difference between total and dissolved aluminum.
It is important to note that coagulant under-dosing can result in substantial deterioration of pathogen removal capability (Huck et al., 2001) and can have the opposite effect to that expected (i.e., it increases the residual aluminum concentration) (MWH, 2012). Thus, it is critical that efforts to minimize residual aluminum concentrations do not compromise the effectiveness of pathogen log removal capability or interfere with the removal of NOM (i.e., disinfection by-product precursors).
Following treatment, the pH should be re-assessed and adjusted accordingly to an acceptable level to minimize corrosion and maintain chemical stability in the distribution system (see Section 4.3) (Health Canada, 2015).
4.2.1.2 Orthophosphate
Orthophosphate added after coagulant addition, either during the rapid mix or immediately ahead of filtration, has been identified as a possible strategy to decrease aluminum residuals, because it can form aluminum-phosphate precipitates that can be removed by filtration (Frommel et al., 2004; Wang et al., 2012b; Health Canada, 2018a). This approach may generate competing chemical reactions during water treatment. Depending on water quality conditions, aluminum-phosphate precipitates are formed, resulting in the loss of orthophosphate for corrosion control, and/or the phosphorus introduces a negative charge to aluminum hydroxide flocs, resulting in poor filtration. The reactions are pH dependent and thus will be source-specific (Edzwald, 2018).
Water utilities evaluating the use of orthophosphate should determine whether lower aluminum residuals are the outcome of a decrease in pH caused by the addition of phosphorus (e.g., would another pH adjusting chemical achieve the same result). This will allow the water utility to conduct a cost-benefit analysis while having regard to the cautions reported in the literature (see below).
Caution is recommended when using orthophosphate for corrosion control, as aluminum can interfere with the passivation of lead (Cantor, 2017). Theoretical solubility models for the lead carbonate-orthophosphate system typically assume the formation of hydroxypyromorphite (Pb5(PO4)3OH), but orthophosphate may precipitate with residual aluminum (range of 29-110 μg/L for this study), forming porous aluminum- and phosphorus-rich deposits that adhere poorly to pipe surfaces and do not effectively inhibit lead release (Wasserstrom et al., 2017). Cantor (2017) reviewed the phosphate-based corrosion control strategies for 12 municipal and non-municipal water systems using ground and surface water and found a strong association between particulate aluminum and the release of particulate lead and copper. Aluminum-phosphate precipitates can also contribute to distribution system deposits (see Section 4.3), turbidity and milky-white colour at the point of use. A target maximum aluminum concentration of 0.050 mg/L is recommended for both the entry point to the distribution system and within the distribution system to avoid these issues (AWWA, 2011b).
4.2.1.3 Use of certified chemicals with minimal aluminum content
Health Canada commissioned a report to determine the potential aluminum contribution to drinking water from five commercially available types of chemicals certified to NSF International (NSF)/American National Standards Institute (ANSI)/National Standard of Canada (CAN) Standard 60 (NSF/ANSI/CAN, 2018). The following commercial chemicals were assessed: ammonium sulphate (chloramination), calcium hypochlorite (disinfectant), calcium hydroxide (pH adjustment), calcium oxide (pH adjustment), and sodium silicate (corrosion control). Data were compiled from product certification and ongoing surveillance evaluations conducted between 2016 and 2017 (NSF, 2018).
The aluminum concentrations measured in the chemical product types are summarized in Table 9. From these results, estimates of the amount of aluminum added to drinking water at the maximum use level of the product can be calculated (i.e., normalized). These normalized results are summarized in Table 10. It is important to note that these are estimated concentrations, not actual concentrations measured in treated drinking water.
A review of the data in Table 10 indicates that a facility adding both calcium hydroxide and sodium silicate could add up to 51 μg/L of aluminum to the drinking water. Although these values are significantly lower than the single product allowable concentration for aluminum specified in NSF/ANSI/CAN Standard 600 (2019a), they may result in the accumulation of aluminum in the distribution system (see Section 4.3). To minimize the amount of aluminum added to treated water, the aluminum content and the maximum anticipated dose that will be applied at the treatment facility should be considered when specifying chemical products. It is necessary to request the aluminum content when specifying chemical products because aluminum is not normally tested by certifying bodies as part of product certification or ongoing surveillance evaluations (Randall, 2020).
Chemical product type | No. detects/ samples | Minimum (mg/kg) |
Median (mg/kg) |
95th percentile (mg/kg) |
Maximum (mg/kg) |
---|---|---|---|---|---|
Ammonium sulphate | 0/25 | 0 | 0 | 0 | 0 |
Calcium hypochlorite | 21/23 | 121 | 223 | 437 | 485 |
Calcium hydroxide | 30/31 | 0.6 | 11 | 33 | 93 |
Calcium oxide | 25/27 | 0.1 | 1.0 | 22 | 30 |
Sodium silicate | 31/36 | 46 | 99 | 392 | 550 |
Chemical product type | No. detects/ samples | Minimum (μg/L) |
Median (μg/L) |
95th percentile (μg/L) |
Maximum (μg/L) |
---|---|---|---|---|---|
Ammonium sulphate | 0/25 | 0 | 0 | 0 | 0 |
Calcium hypochlorite | 21/23 | 0.7 | 2.5 | 5.8 | 6.1 |
Calcium hydroxide | 30/31 | 0.7 | 8 | 21 | 28 |
Calcium oxide | 25/27 | 0.3 | 2.6 | 11 | 15 |
Sodium silicate | 31/36 | 1.9 | 3.9 | 15 | 23 |
4.2.1.4 Other treatment options for naturally occurring aluminum
There is a paucity of literature regarding technologies other than coagulation for the removal of naturally occurring aluminum. Aluminum is known to foul reverse osmosis membranes (Allenby, 2004); cation exchange resins must be modified and used at an extremely low pH (Vanloot et al., 2007); lime softening may increase aluminum concentrations (Reijnen et al., 1991; Alabdula'aly, 1998; Kettunen and Keskitalo, 2000; AWWA, 2011b); and chemical oxidants, such as chlorine, are ineffective because the oxidation state of aluminum does not change (Edzwald, 2018). Based on the occurrence data presented in Table 2, 90th percentile concentrations are below the MAC, while some maximum values are above it. For sources with aluminum concentrations above the MAC, a site-specific assessment would be necessary to determine the most appropriate treatment option if coagulation is not feasible. Pilot testing is essential to ensure the source water can be successfully treated. Alternatively, a safe alternate drinking water supply could be used.
4.2.1.5 Use of activated alumina
Facilities that use activated alumina to remove other contaminants, such as arsenic or fluoride, should be aware that total aluminum concentrations generally increase following treatment (Bellen et al., 1985; Simms and Azizian, 1997; Wang et al., 2000; Lipps et al., 2006; Valigore et al., 2007, Westerhoff et al., 2008; George et al., 2010). Concentrations above the MAC and operational guidance (OG) have been reported in full-scale studies as noted in Table 11. AWWA (2011a) suggests pH adjustment to 5.5-6 to maximize the adsorption capacity of the activated alumina bed, however, this low pH can increase aluminum solubility and result in elevated concentrations (see Figures 1 and 2). In contrast, Valigore et al. (2007) demonstrated that the amount of aluminum added to treated water can be kept low at near neutral pH (i.e., close to the pH of minimum solubility) (see Table 11). Hao and Huang (1986) also noted that pH plays a role in the dissolution of activated alumina media. High concentrations were measured in two of the full-scale studies listed in Table 11 following media regeneration or replacement (see table notes).
Reference | System type | pH | Total aluminum (mg/L) | ||
---|---|---|---|---|---|
Raw water (average) | Treated water | ||||
Average | Maximum | ||||
Bellen et al., 1985 | Small community | Adjusted to 5.5 | Not given | Results were graphed - most were below 0.5 mg/L with some excursions between 0.5-1.5 mg/LTable 11 footnote a | |
Bellen et al., 1985 | Small community | Adjusted to 5-6 | Not given | Results were graphed - most were below 0.25 mg/L with some excursions between 0.25-1 mg/L and end of run concentrations between 1 and 2 mg/L | |
Wang et al., 2000 | Semi-public water supplyTable 11 footnote 1 | Ambient pH = 7.4-8.7 | 0.014 | 0.032 | 0.112 |
Wang et al., 2000 | Semi-public water supplyTable 11 footnote 1 | Ambient pH = 7.7-8.7Table 11 footnote b | 0.015 | 0.040 | 0.190Table 11 footnote b |
Lipps et al., 2006 | Semi-public water supplyTable 11 footnote 1 | Ambient pH = 7.6-8.6 | 0.013 | 0.037 | 0.132 |
Valigore et al., 2007 | Small community | Ambient pH = 7.4-7.8 | <10 | <0.010 | 0.018 |
As pH impacts the release of aluminum from activated alumina media, it is important to select an appropriate pH that considers both the contaminant that is being removed and the amount of aluminum that is added to the treated water (EFSA, 2006; George et al., 2010). Also, an appropriate media should be selected to minimize the residual aluminum concentration (EFSA, 2006). Facilities using activated alumina for drinking water treatment should purchase media that is certified as meeting NSF/ANSI/CAN Standard 61 (2019b) and request aluminum leaching results as part of the purchasing process (Randall, 2020).
Full-scale studies presented in Table 11 demonstrate that, on average, the OG can be achieved. Pilot- and full-scale studies have also found that maximum total aluminum concentrations can be maintained below 0.100 mg/L if pH is near neutral (Simms and Azizian, 1997; Valigore et al., 2007). However, some researchers have observed that depending on the pH and fluoride concentration, alumino-fluro complexes may form and result in higher aluminum concentrations (Hao and Huang, 1986; George et al., 2010). Thus, the responsible drinking water authority in the affected jurisdiction should be contacted to confirm the requirements that will apply to facilities using activated alumina. Monitoring requirements for both the contaminant that is being removed and residual aluminum should also be established.
Bench- or pilot-scale testing is essential to ensure the source water can be successfully treated while minimizing residual aluminum concentrations. Media regeneration should consider the contaminant that is being removed and the aluminum concentration (George et al., 2010). The handling and disposal of residuals generated by activated alumina processes should also be considered (see Section 4.4).
4.2.2 Residential-scale treatment
In cases where aluminum removal is desired at the household level (for example, when a household obtains its drinking water from a private well) treatment is expected to be challenging, based on the information presented in Section 4.2.1. The responsible drinking water authority in the affected jurisdiction should be contacted to discuss possible options.
Residential-scale water supplies that use activated alumina should refer to Section 4.2.1.5 and contact the responsible drinking water authority in the affected jurisdiction to confirm the requirements that will apply, including monitoring.
4.3 Distribution system considerations
4.3.1 Aluminum deposition and accumulation
Observations of aluminum deposits on distribution system piping have been reported in the literature since 1953 (Baylis, 1953; Hudson, 1966; Cooper and Knowles, 1975; Foley, 1980; Costello, 1984; Kriewall et al., 1996; Halton, 2001; Muylwyk and MacDonald, 2001; Schock and Holm, 2003; Lytle et al., 2004; Schock, 2005; Friedman et al., 2010; Grigg, 2010; Li et al., 2018). Aluminum can accumulate on all pipe materials (Hudson, 1966; Halton, 2001; Carrière et al., 2005) and be released, along with other health-based contaminants, when water quality conditions change (e.g., pH or temperature) (Fuge et al., 1992; Kriewall et al., 1996; Halton, 2001; Snoeyink et al., 2003; Kim et al., 2011; Trueman and Gagnon, 2016). Physical/hydraulic disturbances may also cause poorly adhered deposits to detach (e.g., road work, hydrant flushing, watermain breaks, meter installation, leak repair, firefighting activity) (Friedman et al., 2010; Hill et al., 2010; Del Toral et al., 2013; Wasserstrom et al., 2017). Additionally, changes in pH and temperature in the distribution system can cause aluminum to go in and out of solution and be transported and deposited throughout the system (Driscoll et al., 1987; Halton, 2001; Snoeyink et al., 2003; Munk and Faure, 2004).
Table 12 quantifies the accumulation of aluminum in various system types. The majority of results presented in Table 12 are for groundwater systems, which tend to have lower aluminum concentrations. Nonetheless, Lytle et al. (2004) and Friedman et al. (2010) reported 90th percentile aluminum concentrations in pipe section solids that were comparable in groundwater and surface water systems, whereas maximum concentrations were 5.5 and 1.8 times higher in groundwater systems than in surface water. Although surface water data are limited, these data demonstrate that aluminum accumulates in all water systems.
With respect to hydrant flush solids (see Table 12), Lytle et al. (2004) reported the highest aluminum concentration (144 265 μg/g) in a groundwater system with alum addition. This system also had the highest copper, lead and nickel concentrations. In groundwater systems with no alum addition, the maximum aluminum concentration was 19 times lower. Li et al. (2018) measured an aluminum concentration of 55 000 μg/g for unidirectional flush solids from a cast-iron pipe for a surface water system adding PACl (average aluminum residual = 0.050 mg/L). The authors reported that aluminum (and manganese) contribute to the formation of loose deposits that are more easily released by hydraulic disturbances.
Deposit type | Water type | No. of samples | Min (μg/g) |
Median (μg/g) |
90th (μg/g) |
Max (μg/g) |
---|---|---|---|---|---|---|
Pipe section solids | Lytle et al., 2004Table 12 footnote a | |||||
Groundwater | 35 | 28 | 718 | 2 789 | 7 286 | |
Surface water with alum addition | 1 | 1 324 | 1 324 | 1 324 | 1 324 | |
Friedman et al., 2010Table 12 footnote b | ||||||
Groundwater | 22 | 105 | 536 | 3 294 | 8 880 | |
Mixed-groundwater and surface waterTable 12 footnote cc | 8 | 374 | 1 422 | 8 322 | 20 256 | |
Mixed-surface waterTable 12 footnote dd and groundwater | 3 | 561 | 759 | 944 | 990 | |
Surface water with alum addition | 2 | 4 373 | 4 669 | 4 906 | 4 965 | |
Hydrant flush solids | Lytle et al., 2004Table 12 footnote a | |||||
Groundwater | 22 | 96 | 375 | 2 905 | 7 512 | |
Groundwater with alum addition | 4 | 11 708 | 103 602 | 139 252 | 144 265 | |
Friedman et al., 2010Table 12 footnote b | ||||||
Groundwater | 21 | 33 | 446 | 1 066 | 1 659 | |
Mixed-groundwater and surface waterTable 12 footnote e | 2 | 1 545 | 5 911 | 9 403 | 10 276 | |
Li et al. (2018) discussed the cumulative process of deposit mixtures and suggested that aluminum and manganese served as the main scavengers to adsorb other metals. The authors stated that measures to minimize aluminum and manganese deposits in the distribution system were essential to reduce heavy metal-related risks. On average, aluminum ranked eighth out of 13 elements (manganese was seventh) in terms of deposit concentrations in Lytle et al. (2004), whereas aluminum ranked third out of 12 elements (manganese was fifth) in Friedman et al. (2010). Schock (2005) published metal accumulation in lead service lines and iron pipe scales for a variety of water types. On average, aluminum ranked fourth out of 13 elements (manganese was sixth) in terms of deposit concentrations. These data highlight that aluminum and manganese solids can represent a significant portion of legacy deposits in the distribution system.
Health-based contaminants that have accumulated may be released to distributed water as dissolved or particulate species via three mechanisms, namely:
- chemically-influenced processes such as corrosion or adsorption/desorption from materials/deposits in the distribution system (AWWA, 2011b);
- microbially-influenced processes (e.g., biostability) (Cantor, 2017; Health Canada, 2020b); or
- physical/hydraulic changes (Friedman et al., 2010, 2016; Cantor, 2017, 2018; Hill et al., 2018).
When using pH-dependent strategies to control chemically-influenced processes in the distribution system, it is important to recognize that some contaminants are released in response to a pH decrease (e.g., lead, manganese) while others are released in response to a pH increase (e.g., arsenic, copper, chromium) (Kim et al., 2011). A minimum pH of 7.0 is recommended to control corrosion and minimize the release of health-based contaminants; however, a higher pH is often necessary (Health Canada, 2015).
Also, aluminum solubility characteristics can vary seasonally due to changes in temperature, pH and NOM concentrations. Higher temperatures in the summer, for example, may allow aluminum to stay in dissolved form and not precipitate. If the temperature increase is high enough to cause the system to experience subsaturation conditions, previously accumulated aluminum deposits (i.e., legacy deposits) can dissolve and release co-precipitated contaminants. Seasonal variations in other parameters (e.g., phosphate, silicate) can also impact chemical equilibrium processes.
Thus, a comprehensive control strategy that considers both chemical and biological stability, as well as physical/hydraulic disturbances (see Section 5) is required to meet concomitant water quality goals related to aluminum and other health-based contaminants that may accumulate in the distribution system (Cantor, 2017, 2018; Li et al., 2018). Additional guidance regarding pH is available in the Guidelines for Canadian drinking water quality - Guideline technical document: pH (Health Canada, 2015) and for other aspects in the Guidance on monitoring the biological stability of drinking water in distribution systems (Health Canada, 2020b).
4.3.2 Leaching of aluminum from cement-based materials
Aluminum may enter the distribution system through leaching from cement-based reservoirs (Halton, 2001; Ontario Ministry of the Environment and Climate Change, 2017), as well as materials and linings (Leroy et al., 1996) even when using certified materials and linings applied according to industry standards (US EPA, 2002).
Mlynska and Zielina (2017) conducted a bench-scale study to compare the aluminum leaching from two pipe specimens coated with different cement linings: a prefabricated pipe cement coating and a coating prepared onsite during a pipe renovation. Both pipe specimens were filled with water collected from a water treatment plant (aluminum concentration not reported). Water samples were collected from each pipe specimen following specific periods of time for up to 56 days. At the end of the experiment, the aluminum concentrations were approximately 0.03 mg/L and 8 mg/L in the pipe specimen with the prefabricated pipe coating and in the onsite applied coating specimen, respectively. However, it is important to note that this study represents stagnation conditions that generally do not occur in distribution systems. At full-scale testing, Zielina et al. (2015) reported the leaching of aluminum after the application of a cement-mortar lining inside a 500 mm steel pipe (length 614.5 m). Aluminum concentrations increased from 0.043 mg/L to 0.293 mg/L after three hours and decreased to 0.052 mg/L after 11 hours. Berend and Trouwborst (1999) reported aluminum concentrations of 650 μg/L six weeks after 2 200 metres of ductile iron pipe was coated with a cement-mortar lining.
Given that aluminum concentrations can increase when lining watermains onsite with cement mortar, it is recommended that: 1) water utilities follow procedures outlined in ANSI/AWWA Standard C602 (AWWA/ANSI, 2017) and 2) water quality monitoring be conducted to assess whether aluminum is leaching into the drinking water. If the total aluminum concentration is above the MAC, the watermain should be flushed and retested. Additional guidance regarding the leaching of aluminum from cement-based materials and linings is available in the Distribution system issue paper –- Permeation and leaching (US EPA, 2002).
4.4 Residuals management
Treatment technologies may produce a variety of residuals that contain aluminum (e.g., backwash water, reject water/concentrate, media regeneration waste). If residuals are discharged directly to a water body or if the residuals treatment process involves a discharge to a water body, the responsible drinking water authority in the affected jurisdiction should be contacted to confirm the requirements that will apply. Guidance can be found elsewhere (CCME, 2003, 2007).
In some cases, aluminum-rich residual streams (e.g., filter backwash, thickener supernatant) are recycled to the head of the treatment plant to improve water recovery rates. Where feasible, these streams should be treated prior to the recycling to remove solids (including aluminum particles and co-precipitated contaminants), improving and stabilizing the treated water quality (Confluence Engineering, 2018). Recycled residual streams should, in all cases, be treated prior to recycling to reduce risks from concentrated enteric protozoa and viruses (Health Canada, 2019a, 2019b). The responsible drinking water authority in the affected jurisdiction should be contacted to confirm applicable requirements.
5. Management strategies
All water utilities should implement a risk management approach, such as the source-to-tap or water safety plan approach, to ensure water safety (CCME, 2004; WHO, 2011a, 2012). These approaches require a system assessment to characterize the source water, describe the treatment barriers that prevent or reduce contamination, identify the conditions that can result in contamination, and implement control measures. Operational monitoring is then established, and operational/management protocols are instituted (e.g., standard operating procedures, corrective actions and incident responses). Compliance monitoring is determined and other protocols to validate the water safety plan are implemented (e.g., record keeping, consumer satisfaction). Operator training is also required to ensure the effectiveness of the water safety plan at all times (Smeets et al., 2009).
5.1 Control strategies
As it is difficult to control the accumulation and release of aluminum and other health-based contaminants in the distribution system, the control strategy should minimize the aluminum concentration that enters the distribution system from the treatment plant. AWWA (2011c) recommends that facilities using aluminum-based coagulants aim to restore total aluminum concentrations to less than or equal to raw water concentrations or achieve 0.050 mg/L in treated water. Secondly, the distribution system should be managed such that drinking water is transported from the treatment plant to the consumer with minimum loss of quality. As source waters, treatment plants and distribution systems can differ significantly, a system-specific control strategy would be necessary.
Municipal, semi-public or residential-scale water supplies using activated alumina for the removal of other contaminants (e.g., arsenic, fluoride) should contact the responsible drinking water authority in the affected jurisdiction to confirm the applicable requirements.
5.1.1 Treatment
There is extensive guidance available to assist water utilities in understanding the mechanisms associated with coagulation (Edzwald, 1993; Pernitsky, 2003; Dempsey, 2006; O'Melia, 2006; Pernitsky and Edzwald, 2006; Shin et al., 2008; Edzwald and Kaminski, 2009; AWWA, 2011c; Davis and Edwards, 2014). Jar testing is preferred for optimization studies, as it is relatively easy to perform experiments using various coagulant types, dose, pH, and mixing speeds.
The most common strategies for decreasing the residual aluminum concentration include: (i) optimizing the pH of coagulation; (ii) using a pre-hydrolyzed coagulant (e.g., PACl, aluminum chlorohydrate, polyaluminum sulphate, polyaluminum silica sulphate); or (iii) using an iron-based coagulant. For direct filtration treatment plants and other facilities that have short hydraulic detention times or changing water quality, other options such as the addition of a clarification process may need to be considered (Driscoll and Letterman, 1988; Halton, 2001; Valade et al., 2009). Combining aluminum- and iron-based coagulants has also been studied (Geng, 2005; Kazza, 2015; Elliott et al., 2016).
Optimum pH ranges for coagulation are noted in Table 5 (see Section 4.2). When implementing pH control, water utilities should be aware that as pH increases, NOM becomes more negatively charged and coagulant hydrolysis products becomes less positively charged (Edzwald, 1993). As a result, when seasonally adjusting the coagulation pH, the coagulant dose must be reviewed and adjusted accordingly (Duan and Gregory, 2003). Otherwise, the coagulant can be under- or over-dosed (depending on the season) and turbidity can increase (Sharp, 2015). In addition, the impact of post-chlorination on pH (e.g., decrease with chlorine gas or increase with sodium hypochlorite) should also be assessed, particularly if the dose is adjusted on a seasonal basis (Larson and Sollo, 1967; Costello, 1984; Reijnen et al., 1991).
When selecting a coagulant dose, water utilities should be aware that under-dosing to attempt to decrease the aluminum residual can result in substantial deterioration of the pathogen removal capability (Huck et al., 2001) and can have the opposite effect to that expected (i.e., it increases the residual aluminum concentration) (MWH, 2012). Adequate coagulant dosing and strict pH control are necessary to practice optimum coagulation and minimize residual aluminum. It is also important to consider alkalinity when applying coagulants. When coagulants are added to water, alkalinity is consumed and carbon dioxide is released which decreases the pH (AWWA, 2011a). The amount of alkalinity consumed varies by coagulant. Pre-hydrolyzed forms of aluminum (e.g., polyaluminum coagulants) consume less alkalinity as they are pre-neutralized and therefore less acidic (see Section 4.2). Generally, alkalinity is consumed by coagulants in the following order: aluminum chlorohydrate ≈ polyaluminum silica sulphate < PACl < polyaluminum sulphate < alum ≈ ferric sulphate < ferric chloride. However, jar tests should be conducted to confirm the effect on alkalinity and pH for the water being treated. Jar testing is recommended because alkalinity is measured by titrating to a specific endpoint pH during the laboratory analysis (APHA et al., 2017). For drinking water samples, an endpoint pH of 4.5 is typically used. As water is not coagulated at such a low pH, jar tests provide useful information to optimize coagulation conditions and for post-coagulation pH adjustment to maintain chemical stability in the distribution system (see Section 4.3) (Health Canada, 2015). The monitoring of other parameters for process control (e.g., temperature, UV254, zeta potential) can also optimize performance.
Optimizing the pH of coagulation is not always practiced (Kimura et al., 2013). In some cases, water sources are alkaline and a depression in pH must be followed by an increase in pH for corrosion control. In other cases, water utilities may choose to coagulate at a lower pH to minimize the negative charge on NOM and maximize the positive charge on coagulant hydrolysis products. For alkaline sources (pH >7), the use of pre-hydrolyzed coagulants (e.g., PACl) can decrease the residual aluminum concentration entering the distribution system (Kimura et al., 2013). Results vary based on coagulant basicity and other source-specific variables (e.g., turbidity, NOM). Kimura et al. (2013) reported that basicity >70% is essential to decrease residual aluminum concentrations. For facilities that coagulate at lower than optimum pH for NOM removal, studies have found that iron-based coagulants can be more effective than aluminum-based coagulants (Budd et al., 2004; AWWA, 2011c; Sillanpää and Matilainen, 2015). The most effective pH range is 4.5-6 as iron hydrolysis products have little positive charge above 6.5.
In all cases, source-specific treatability studies should be conducted to identify the most effective coagulant and the optimal conditions that will maximize turbidity/NOM removal and minimize the residual aluminum concentration. The lack of a source-specific treatability study may result in the selection of inappropriate treatment, an increase in corrosion potential or other unintended consequences.
5.1.2 Distribution system
There is increasing recognition that distribution systems represent a complex and dynamic environment, where numerous interactions and reactions capable of impacting aluminum concentrations at consumer taps can occur. Seasonal source water quality fluctuations (including temperature), process control modifications or other causes can ultimately affect the fate and transport of aluminum in the distribution system, resulting in an increase in aluminum concentrations at the tap. Other events or water utility practices may also result in water chemistry changes (e.g., blending of different sources, nitrification) (Hill et al., 2010).
To minimize the degradation of water quality, water utilities should maintain stable water chemistry conditions that promote consistent equilibrium-based solubility of aluminum, preferably subsaturation to reduce the risk of precipitation and the accumulation of aluminum throughout the distribution system. Stable water chemistry conditions also minimize the risk of desorption (release) of aluminum and co-occurring health-based contaminants that can be complexed or co-precipitated on or within the legacy aluminum deposits (as well as manganese deposits or other solids). Key water quality parameters relevant to these mechanisms include pH, temperature, oxidation-reduction potential, NOM, sulphate, dissolved inorganic carbon, fluoride, and residual concentrations of orthophosphate or silicate (when applied for corrosion control). In addition, water quality that is non-aggressive towards concrete and cement pipe types and cement-mortar linings should be maintained to minimize leaching of aluminum (and calcium, etc.) from these matrices (Leroy et al., 1996). Water utilities should determine the baseline water quality entering and within their distribution systems and subsequently establish boundary conditions outside of which an excursion could be expected to trigger a release event (Friedman et al., 2016).
Depending on the situation, there are a variety of methods to improve the stability of these parameters, such as installation of treatment, modification of existing treatment processes, enhanced process monitoring and control (at the treatment plant and/or in the distribution system), slow and controlled introduction of new or seasonal sources, and controlled blending of dissimilar sources (Confluence Engineering, 2018). Prior to the introduction of a new source and/or the application of a new or modified treatment process for an existing source, the responsible drinking water authority in the affected jurisdiction should be contacted to confirm applicable requirements. Pilot testing should be conducted using harvested pipe specimens from the system to consider the following points and avoid unintended consequences (Hill and Giani, 2017):
- assess the occurrence and inventory of aluminum and other health-based contaminants in the pipe scales;
- identify a pipe and deposit/scale response to the new source or water chemistry; and
- evaluate approaches to mitigate any observed adverse responses.
When selecting an appropriate pH for distributed water, chemically-influenced processes (i.e., corrosion, adsorption/desorption) should be considered to minimize the accumulation and release of health-based contaminants (see Section 4.3.1). A minimum pH of 7.0 is recommended although a higher pH is often necessary (Health Canada, 2015). Distribution system pH variability should also be minimized to ±0.2 units (Muylwyk and MacDonald, 2001; Friedman et al., 2010; Health Canada, 2015).
Biostability in the distribution system is another important requirement to minimize contaminant accumulation and release. Biostability can be achieved by minimizing nutrients in the water (e.g., organic carbon, ammonia, nitrate/nitrite, total phosphorus), managing water age and maintaining a sufficient disinfectant residual (Cantor, 2017; Health Canada, 2020a, 2020b). Strategies to minimize physical and hydraulic disturbances should also be developed (Health Canada, 2020b).
Other measures that contribute to maintaining stable chemical and biological conditions in the distribution system include pipe cleaning (e.g., unidirectional flushing, pipe pigging), pipe replacement, and appropriate treatment to minimize the loading of other contaminant sinks (e.g., iron, manganese) and decrease the concentrations of contaminants entering the distribution system (e.g., arsenic, barium, chromium, manganese) (Friedman et al., 2010; Cantor, 2017).
For systems that use orthophosphate for corrosion control, the orthophosphate should be applied at all system entry points and a consistent residual concentration should be maintained throughout the distribution system to promote the stability of phosphate-based scales (Friedman et al. 2010). It should be noted that polyphosphates (i.e., blended ortho/poly products) can soften cementitious matrices and leach aluminum (and calcium, etc.) into the distribution system (Leroy et al., 1996).
5.1.3 Buildings/premise plumbing
Building owners or managers have the responsibility to monitor and manage their water systems to ensure safe water at the consumers' tap. Although it is beyond the scope of this document to specify where and when to routinely monitor building/premise plumbing, it is recommended that a flushed sample be collected to monitor total aluminum concentrations. Results should be compared to the MAC and OG (e.g., to assess if aluminum is influencing the concentration of co-occurring health-based contaminants such as lead or copper), as well as concentrations measured at the treatment plant and distribution system by the water utility. The latter results can be requested from the water utility if they are not publicly available. If an exceedance of the MAC or OG is reported, building owners or managers should contact the responsible drinking water authority in the affected jurisdiction.
In addition, building owners or managers should be aware that water utilities conduct watermain flushing to remove sediment and accumulated deposits from their distribution systems (see Section 4.3.1) or to manage other water quality issues (Locco et al., 2018). Watermain flushing may cause discoloured water to enter the building service line. Thus, after the flushing is completed, cold water taps should be opened to eliminate any discoloured water that may have entered (Locco et al., 2018). Discoloured water should not be considered safe to consume until it has been tested and confirmed to be safe (Friedman et al., 2016).
More information on managing water safety in buildings is available elsewhere (WHO, 2011b; Health Canada, 2013).
5.2 Monitoring
Aluminum concentrations can vary in source water and within treatment plants and distribution systems; therefore, monitoring programs should be established that enable water utilities to obtain a good understanding of aluminum concentrations from source to tap. Monitoring programs should be designed to verify that control strategies are operating as intended and to consider risk factors that contribute to the likelihood of aluminum being elevated within the drinking water system.
Monitoring of aluminum concentrations is intended to apply to all water systems, including groundwater systems (AWWA, 2015). The literature indicates that aluminum accumulates in all water systems (Lytle et al., 2004; Schock, 2005; Friedman et al., 2010). In particular, maximum aluminum concentrations in pipe section solids in groundwater systems were found to be 5.5 and 1.8 times higher than in surface water systems even though groundwater sources tend to have lower aluminum concentrations than surface water (see Section 4.3.1). Furthermore, a survey of distribution system cleaning practices found that groundwater systems generate sediment (e.g., aluminum silicate) that must be flushed from the system (Halton, 2001). The proper development of groundwater wells, during the construction phase, is a key element to minimize sediment generation and maximize well efficiency (Ontario Ministry of Agriculture, Food and Rural Affairs, 1997). However, even wells that produce water with turbidity <1 NTU and that have been developed to industry standards, have been shown to produce sediment following rest periods, during start-up and from discrete intervals during routine pumping. This may lead to the gradual accumulation of sediment in the distribution system, storage facilities, etc. (Lotimer, 2012).
Municipal, semi-public or residential-scale water supplies using activated alumina for the removal of other contaminants (e.g., arsenic, fluoride) should contact the responsible drinking water authority in the affected jurisdiction to confirm monitoring requirements.
5.2.1 Source water characterization
Source water characterization should be part of routine system assessments and should include an understanding of aluminum concentrations in the source water (both groundwater and surface water) and conditions that can lead to changes in these concentrations. Source water monitoring should be conducted in conjunction with other monitoring, as discussed below.
5.2.2 Operational monitoring
As aluminum is an important process parameter to practice optimum coagulation, water utilities that use aluminum-based coagulants should conduct daily or more frequent monitoring of total aluminum. These measurements should be conducted onsite using an online or portable colorimetric analyzer. An appropriate QA/QC and verification program should also be in place. To minimize interferences, samples can be collected after filtration before any fluoride or phosphate addition. Monitoring of dissolved aluminum concentrations is also recommended for process control. AWWA (2011c) recommends that water utilities that use aluminum-based coagulants should aim to restore total aluminum concentrations to less than or equal to raw water concentrations or achieve 0.050 mg/L in treated water. Thus, raw water monitoring is necessary.
Measures should also be in place to assess the contribution of aluminum from other water treatment chemicals. This can be determined by comparing aluminum concentrations in the filter effluent and treated water when aluminum-based coagulants are used or by comparing raw and treated aluminum concentrations for other systems.
The best approach for calculating the locational running annual average for comparison with the OG value is as follows:
- For facilities that use aluminum-based coagulants:
- At the entry point to the distribution system (or before fluoride/phosphate addition) - based on daily samples that are averaged on a monthly basis, these monthly averages should then be used to calculate a locational running annual average. This will ensure that seasonal trends that result in elevated aluminum residuals are appropriately captured in the running annual average.
- For facilities that do not use aluminum-based coagulants:
- At the entry point to the distribution system - based on a locational running annual average of a minimum of quarterly samples.
The responsible drinking water authority in the affected jurisdiction should be contacted to confirm requirements. The responsible authority may require: 1) more frequent monitoring during facility upsets, highly variable source water quality conditions or other conditions; or 2) less frequent monitoring during stable water quality conditions.
5.2.3 Distribution system monitoring
Given that aluminum concentrations can change throughout the distribution system (Halton, 2001), appropriate distribution system monitoring should be conducted (Friedman et al., 2010) in conjunction with paired source- and treated-water sampling. It is recommended that aluminum concentrations be measured in free-flowing (e.g., flushed) samples. Samples should be collected at critical points within the distribution system; a sufficient number of sites should be sampled to be representative of the population served and the hydraulic zones within the distribution system. Areas with variable turbidity (e.g., particulate solids in the water) or conductivity (e.g., dissolved solids in the water) should be targeted. Turbidity and conductivity are general water quality parameters indicating changes in deposit accumulation and release (Cantor, 2018). In the absence of turbidity and conductivity data, sites where bacterial indicator samples are collected can be used in the interim. Sample locations can be refined as water quality data is collected and trends are assessed. Monitoring the inlet and outlet of concrete reservoirs is also suggested to ensure that water quality is not aggressive to these facilities and thereby leaching aluminum into distributed water (Halton, 2001).
Monitoring should include dissolved and total aluminum concentrations, pH, temperature, and orthophosphate residual (if relevant) (Cantor, 2017). Knowing whether aluminum is present in dissolved versus particulate form is helpful to assess the fate and transport of aluminum within the distribution system and to diagnose potential mechanisms leading to upsets or release events. Specifically, an increase in particulate aluminum concentrations (relative to the treatment facility or upstream sample locations) is usually indicative of a hydraulic release or destabilization event in which aluminum precipitates or aluminum-laden deposits are stirred up and entrained. Depending on the magnitude and frequency of the increase, this may suggest the need for watermain cleaning (e.g., unidirectional flushing) to remove hydraulically-mobile deposits. In contrast, an increase in dissolved aluminum concentrations may be indicative of a disequilibrium-based chemical release (e.g., dissolution or desorption). This may require the water utility to adjust or achieve tighter control over treated water quality (e.g., pH, phosphate); water age or temperature management may also be necessary. Chronic, elevated dissolved aluminum concentrations in the distribution system (relative to the treatment facility) may also be due to water that is overly aggressive towards cement-mortar lining, concrete pipes or reservoirs, in which case treatment adjustments may be needed.
A locational running annual average of a minimum of quarterly samples should be calculated for comparison with the MAC. With respect to the OG value, the best approach for calculating the locational running annual average is as follows:
- For facilities that use aluminum-based coagulants:
- Within the distribution system - based on a locational running annual average of a minimum of monthly samples.
- For facilities that do not use aluminum-based coagulants:
- Within the distribution system - based on a locational running annual average of a minimum of quarterly samples.
To minimize the potential for the accumulation and release of aluminum and co-occurring contaminants, for interference with orthophosphate (where applicable) and for aesthetic issues (e.g., colour, turbidity), water utilities should strive to maintain aluminum concentrations below 0.050 mg/L throughout the distribution system (AWWA, 2011b, 2011c).
In addition, event-based monitoring should be conducted during conditions where the risk of release is increased, such as following hydraulic disturbances (e.g., watermain flushing) or changes in water chemistry (e.g., changes to pH, temperature, source water type, chlorine residual) as well as when discolouration of water has been reported (Friedman et al., 2016). Some samples should be collected from sites within the distribution system (such as hydrants or valves) as well as from drinking water taps in public or private buildings to help determine the cause of the event and the aluminum concentrations at the point of use (i.e., tap). Event-based samples should also be analyzed for other metals that can co-occur in the distribution system and be released with aluminum (e.g., arsenic, chromium, copper, iron, lead, manganese, nickel).
The responsible authority may direct that a plan be developed and implemented to address persistent exceedances of any locational running annual averages. Corrective actions may include, but are not limited to: watermain cleaning to remove hydraulically-mobile deposits; treatment process change to address chemical instability; steps to manage water age or temperature impacts.
5.2.4 Compliance monitoring
Total aluminum in drinking water based on a locational running annual average of a minimum of quarterly samples taken in the distribution system should be calculated for comparison with the MAC. However, as with all of the Canadian guidelines for drinking water, any exceedance should be a signal to evaluate the situation, take corrective action(s) and consult with the responsible drinking water authority in the affected jurisdiction. The responsible authority may direct that a plan be developed and implemented to address the situation.
With respect to the OG, the responsible drinking water authority in the affected jurisdiction should be contacted to confirm the applicable requirements for compliance monitoring.
Water utilities that undertake preventive measures with stable hydraulic, physical, chemical and biological water quality conditions and that have baseline data indicating that aluminum does not occur in the system may contact the responsible drinking water authority in the affected jurisdiction to request less frequent monitoring.
5.2.5 Deposit characterization and inventory
There are limited data suggesting that health-based contaminants measured at the tap (e.g., lead) originate from aluminum deposits. More work is required to determine whether these interactions are similar to those between lead and iron in drinking water systems. Characterization of pipe deposits may help in gaining a better understanding of aluminum interactions with other elements. Speciation of aluminum (i.e., particulate and dissolved) and other elements at the point of use may identify pathways by which trace inorganic contaminants are mobilized (e.g., aluminum-rich particulate matter with adsorbed lead). This work involves specialized methods that may require a partnership between water utilities and universities or advanced commercial laboratories.
Establishing the mass inventory (i.e., mass per pipe wall area) of aluminum and other contaminants contained within distribution system deposits is also encouraged to obtain site-specific concentration increases that could occur under a release scenario (Brandhuber et al., 2015). The Friedman et al. (2010) study provides guidance on sampling pipe specimens to establish an inventory of distribution system solids mass and composition.
6. International considerations
Other national and international organizations have drinking water guidelines, standards and/or guidance values for aluminum in drinking water. Variations in these values can be attributed to the age of the assessments or to differing policies and approaches, including the choice of key study and the use of different consumption rates, body weights and source allocation factors.
No other national or international agency has established regulatory limits for aluminum in drinking water based on health considerations. Rather, non-regulatory guidance values have been set based on aesthetic or operational considerations. The WHO has set practicable values of 0.1-0.2 mg/L based on optimization of the coagulation process in drinking water plants (WHO, 2010). The US EPA has set a secondary maximum contaminant level of 0.05-0.2 mg/L (Dietrich, 2015), Australia has chosen an aesthetic objective of 0.2 mg/L (NHMRC and NRMMC, 2011) and New Zealand has a guideline value of 0.1 mg/L for aesthetic considerations (New Zealand Ministry of Health, 2008). The European Union lists aluminum as an indicator parameter in its drinking water directive with a value of 0.2 mg/L (EU, 1998).
In its assessment of aluminum in drinking water, the WHO (2010) calculated a health-based value of 0.9 mg/L (rounded) but has highlighted the importance of not exceeding the practicable levels of 0.1-0.2 mg/L to ensure optimization of the coagulation process, in order to prevent microbial contamination and minimize deposition of aluminum floc in the distribution system. Canada's guideline value differs from the WHO's health-based value because Canada takes into consideration advancements in science since 2010. The WHO assessment is based on the Joint Food and Agriculture Organization of the United Nations/WHO Expert Committee on Food Additives' (JECFA) previous Provisional Tolerable Weekly Intake (PTWI) for aluminum of 1 mg/kg bw per day (JECFA, 2007). JECFA has since revised their PTWI to 2 mg/kg bw per day (JECFA, 2012) based on the key study, Poirier et al. (2011), that is also used in the Canadian guideline.
7. Rationale
The MAC of 2.9 mg/L (2 900 μg/L) is protective of potential health effects and can be reliably measured by available analytical methods and achieved by coagulation. However, the presence of aluminum at low concentrations can cause operational and aesthetic issues in the distribution system. Scientific findings related to the accumulation and release of metals in the distribution system support the need for an OG value; aluminum can also coat watermains, service lines and water meters, resulting in pressure losses, meter malfunctions or turbid/discoloured water. Therefore, an OG of 0.100 mg/L (100 μg/L) for total aluminum is established to avoid these issues. The OG applies to both the entry point to the distribution system and within the distribution system. It is a risk-managed value for the reasons discussed below.
Aluminum is present in drinking water sources both naturally and as a result of human activities. Aluminum concentrations in water vary across Canada, with surface water generally presenting higher concentrations than groundwater. Aluminum salts are commonly added as coagulants during water treatment to remove turbidity, organic matter and microorganisms. Aluminum is also an impurity found in other chemicals used in water treatment and has been found to leach from cement-based materials into drinking water. In addition, aluminum can be added to drinking water as a result of using activated alumina treatment to remove other contaminants (e.g., arsenic, fluoride). Based on aluminum's chemical properties, the intake of aluminum from drinking water is by ingestion and is not expected to occur through either skin contact or inhalation while showering and bathing.
The nervous system is generally considered to be the major target for aluminum toxicity. Studies in animals have consistently observed adverse neurological effects following ingestion of high levels of aluminum, which supports effects seen in human studies. Studies in humans have found possible associations between aluminum ingestion and neurological diseases such as dementia and AD; however, study design limitations preclude the use of these studies as a basis to develop the HBV. The HBV of 2.9 mg/L (2 900 μg/L) for total aluminum is established based on neurological effects observed in rats. The HBV is based on the latest science, and in particular on rigorous studies that were not available for the calculation of previous HBVs (e.g., the WHO's HBV of 0.9 mg/L). For the purposes of this risk assessment, the HBV is designated as the MAC because the HBV is achievable by treatment and reliably measured.
With respect to the OG, the AWWA (2011b, 2011c) recommends a target maximum aluminum concentration of 0.050 mg/L (50 µg/L) for both the entry point to the distribution system and within the distribution system to optimize coagulation and minimize the accumulation and release of health-based contaminants in the distribution system. Despite this, Canadian water utilities have indicated that consistently achieving 0.050 mg/L (50 µg/L) would be challenging due to:
- unintended consequences as a result of pH adjustment or other process changes;
- health and safety issues related to the use of pH adjustment chemicals; and
- economic feasibility.
Based on the above-noted considerations, an OG of 0.100 mg/L (100 µg/L) is established as it will help minimize problems related to the accumulation and release of aluminum and co-occurring contaminants in the distribution system, while remaining achievable for the majority of Canadian water utilities.
The MAC and OG are intended to apply to all water systems, including groundwater systems, as the literature indicates that aluminum accumulates in all water systems.
As part of its ongoing guideline review process, Health Canada will continue to monitor new national and international research in this area and will recommend any change to the guidelines or OG value that is deemed necessary.
8. References
- Abdel Moneim, A., Othman, M.S., Mohmoud, S.M. and El-Deib, K.M. (2013). Pomegranate peel attenuates aluminum-induced hepatorenal toxicity. Toxicol. Mech. Method., 23(8): 624-633.
- Abu-Taweel, G.M., Ajarem, J.S. and Ahmad, M. (2012). Neurobehavioral toxic effects of perinatal oral exposure to aluminum on the developmental motor reflexes, learning, memory and brain neurotransmitters of mice offspring. Pharmacol. Biochem. Be., 101(1): 49-56.
- Akatsu, H., Hori, A., Yamamoto, T., Yoshida, M., Mimuro, M., Hashizume, Y., Tooyama, I. and Yezdimer, E.M. (2011). Transition metal abnormalities in progressive dementias. Biometals, 25(2): 337-350.
- Akinola, O.B., Biliaminu, S.A., Adediran, R.A., Adeniye, K.A. and Abdulquadir, F.C. (2015). Characterization of prefrontal cortex microstructure and antioxidant status in a rat model of neurodegeneration induced by aluminium chloride and multiple low-dose streptozotocin. Metab. Brain Dis., 30(6): 1531-1536.
- Akinola, O.B., Biliaminu, S.A., Adedeji, O.G., Oluwaseun, B.S., Olawoyin, O.M. and Adelabu, T.A. (2016). Combined effects of chronic hyperglycaemia and oral aluminium intoxication on testicular tissue and some male reproductive parameters in Wistar rats. Andrologia, 48(7): 779-786.
- Akinrinade, I.D., Memudu, A.E., Ogundele, O.M. and Ajetunmobi, O.I. (2015). Interplay of glia activation and oxidative stress formation in fluoride and aluminium exposure. Pathophysiology, 22(1): 39-48.
- Akiyama, H., Hosokawa, M., Kametani, F., Kondo, H., Chiba, M., Fukushima, M. and Tabira, T. (2011). Long-term oral intake of aluminium or zinc does not accelerate Alzheimer pathology in ABPP and ABPP/tau transgenic mice. Neuropathology, 32(4): 390-397.
- Alabdula'aly, A. (1998). Trace metals in groundwater and treatment plant product water of the central region of Saudi Arabia. Desalination, 120(1-2): 163-168.
- Alberta Environment and Parks (2017). Personal communication with Donald Reid, Operations Division.
- Albizzati, A., More, L., Di Candia, D., Saccani, M. and Lenti, C. (2012). Normal concentrations of heavy metals in autistic spectrum disorders. Minerva Pediatr., 64(1): 27-31.
- Allenby, M. (2004). Aluminum compounds cause operational problems in a reverse osmosis plant. Mater. Perform., 43(5): 44-46.
- Altmann, P., Cunningham, J., Dhanesha, U., Ballard, M., Thompson, J. and Marsh, F. (1999). Disturbance of cerebral function in people exposed to drinking water contaminated with aluminium sulphate: Retrospective study of the Camelford water incident. BMJ, 319(7213): 807-811.
- Anderson, W.B., Jasim, S.Y., Urfer, D. and Huck, P.M. (1998). Coagulant and pH control alternatives for soluble aluminum control. In: Proceedings of the American Water Works Association Water Quality Technology Conference, San Diego, California. American Water Works Association, Denver, Colorado.
- Anderson, L.E., Krkošek, W.H., Stoddart, A.K., Trueman, B.F. and Gagnon, G.A. (2017). Lake recovery through reduced sulfate deposition: A new paradigm for drinking water treatment. Environ. Sci. Technol., 51(3): 1414-1422.
- APHA, AWWA and WEF (1995). Standard methods for the examination of water and wastewater. 19th edition. American Public Health Association, American Water Works Association and Water Environment Federation, Washington, DC.
- APHA, AWWA and WEF (2017). Standard methods for the examination of water and wastewater. 23rd edition. American Public Health Association, American Water Works Association and Water Environment Federation, Washington, DC.
- Appelo, C.A.J. and Postma, D. (1996). Geochemistry, groundwater and pollution. Third edition. A.A. Balkema, Rotterdam, Netherlands.
- Arain, M.S., Afridi, H.I., Kazi, T.G., Talpur, F.N., Arain, M.B., Kazi, A., Arain, S.A. and Ali, J. (2015). Correlation of aluminum and manganese concentration in scalp hair samples of patients having neurological disorders. Environ. Monit. Assess., 187(2): 10.
- ATSDR (2008). Toxicological profile for aluminum. Agency for Toxic Substances and Disease Registry, Public Health Service, U.S. Department of Health and Human Services, Atlanta, GA.
- AWWA (2011a). Water quality and treatment: a handbook of community water supplies. 6th edition. J.K. Edzwald (ed.). McGraw-Hill, New York, New York.
- AWWA (2011b). Internal corrosion control in water distribution systems: Manual of water supply practices M58. First edition. American Water Works Association, Denver, Colorado.
- AWWA (2011c). Operational control of coagulation and filtration processes: Manual of water supply practices M37. Third edition. American Water Works Association, Denver, Colorado.
- AWWA (2015). Iron and manganese removal handbook, 2nd ed. American Water Works Association, Denver, Colorado.
- AWWA/ANSI (2017). American Water Works Association/American National Standards Institute Standard C602: Cement-mortar lining of water pipelines in place-4 in. (100 mm) and larger. American Water Works Association, Denver, Colorado.
- Bakar, C., Karaman, H., Baba, A. and Sengunalp, F. (2010). Effect of high aluminum concentration in water resources on human health, case study: Biga Peninsula, northwest part of Turkey. Arch. Environ. Contam. Toxicol., 58(4): 935-944.
- Baum, L., Chan, I.H., Cheung, S.K., Goggins, W.B., Mok, V., Lam, L., Leung, V., Hui, E., Ng, C., Woo, J., Chiu, H.F., Zee, B.C., Cheng, W., Chan, M.H., Szeto, S., Lui, V., Tsoh, J., Bush, A.I., Lam, C.W. and Kwok, T. (2010). Serum zinc is decreased in Alzheimer's disease and serum arsenic correlates positively with cognitive ability. Biometals, 23(1): 173-179.
- Baylis, J.R. (1953). Cast-iron pipe coatings and corrosion. J. Am. Water Works Assoc., 45(8): 807-831.
- Belaid-Nouira, Y., Bakhta, H., Bouaziz, M., Flehi-Slim, I., Haouas, Z. and Cheikh, H.B. (2012). Study of lipid profile and parieto-temporal lipid peroxidation in AlCl3 mediated neurotoxicity. Modulatory effect of fenugreek seeds. Lipids Health Dis., 11(1): 16.
- Belaid-Nouira, Y., Bakhta, H., Haouas, Z., Flehi-Slim, I. and Cheikh, H.B. (2013a). Fenugreek seeds reduce aluminum toxicity associated with renal failure in rats. Nutr. Res. Pract., 7(6): 466.
- Belaid-Nouira, Y., Bakhta, H., Haouas, Z., Flehi-Slim, I., Neffati, F., Najjar, M.F. and Cheikh, H.B. (2013b). Fenugreek seeds, a hepatoprotector forage crop against chronic AlCl3 toxicity. BMC Vet. Res., 9(1): 22.
- Belaid-Nouira, Y., Bakhta, H., Samoud, S., Trimech, M., Haouas, Z. and Cheikh, H.B. (2013c). A novel insight on chronic AlCl3 neurotoxicity through IL-6 and GFAP expressions: Modulating effect of functional food fenugreek seeds. Nutr. Neurosci., 16(5): 218-224.
- Bellen, G., Anderson, M. and Gottler, R. (1985). Defluoridation of drinking water in small communities. Prepared for U.S. Environmental Protection Agency, Cincinnati, Ohio. Cooperative Agreement No. R809248010.
- Berend, K. and Trouwborst, T. (1999). Cement-mortar pipes as a source of aluminum. J. Am. Water Works Assoc., 91(7): 91-100.
- Bhasin, P., Singla, N. and Dhawan, D.K. (2012). Protective role of zinc during aluminum-induced hepatotoxicity. Environ. Toxicol., 29(3): 320-327.
- Bhattacharjee, S., Zhao, Y., Hill, J.M., Culicchia, F., Kruck, T.P.A., Percy, M.E., Pogue, A.I., Walton, J.R. and Lukiw, W.J. (2013). Selective accumulation of aluminum in cerebral arteries in Alzheimer's disease (AD). J. Inorg. Biochem., 126: 35-37.
- Brandhuber, P., Craig, S., Friedman, M., Hill, A., Booth, S. and Hanson, A. (2015). Legacy of manganese accumulation in water systems. Water Research Foundation, Denver, Colorado.
- British Columbia Ministry of Health (2017). Personal communication with Drinking Water Manager David Fishwick.
- Callan, A.C., Devine, A., Qi, L., Ng, J.C. and Hinwood, A.L. (2015). Investigation of the relationship between low environmental exposure to metals and bone mineral density, bone resorption and renal function. Int. J. Hyg. Environ. Health, 218(5): 444-451.
- CFIA (2018). Chemical residues in food: Children's food project report 2013-2014 and Arsenic, cadmium, lead, mercury, and aluminum in infant formulas meal replacements, and nutritional supplements 2012-2013. Food Safety Science Directorate, Canadian Food Inspection Agency, Ottawa. Available at: http://www.inspection.gc.ca/food/chemical-residues-microbiology/chemical-residues/eng/1324258929171/1324264923941
- Cantor, A. (2017). Optimization of phosphorus-based corrosion control chemicals using a comprehensive perspective of water quality. Water Research Foundation, Denver, Colorado.
- Cantor, A.F. (2018). Water distribution system monitoring: A practical approach for evaluating drinking water quality. 2nd ed. CRC Press, Boca Raton.
- Cantwell, R.E., Muylwyk, Q., Waller, M., Stuart, J., Rossi, G. and Snoeyink, V.L. (2012). Role of pH for phosphate-based lead control in Windsor, Ontario, Canada. In: Proceedings of the American Water Works Association Water Quality Technology Conference, Toronto, Ontario. American Water Works Association, Denver, Colorado.
- Carrière, A., Gauthier, V., Desjardins, R., & Barbeau, B. (2005). Evaluation of loose deposits in distribution systems through unidirectional flushing. J. Am. Water Works Assoc., 97(9), 82-92.
- CCME (2003). Guidance on the site-specific application of water quality guidelines in Canada: Procedures for deriving numerical water quality objectives. Canadian Council of Ministers of the Environment, Winnipeg, Manitoba.
- CCME (2004). From source to tap: Guidance on the multi-barrier approach to safe drinking water. Canadian Council of Ministers of the Environment, Winnipeg, Manitoba.
- CCME (2007). A protocol for the derivation of water quality guidelines for the protection of aquatic life. Canadian Council of Ministers of the Environment, Winnipeg, Manitoba.
- Cetin, I., Nalbantcilar, M.T., Tosun, K. and Nazik, A. (2017). How trace element levels of public drinking water affect body composition in Turkey. Biol. Trace Elem. Res., 175(2): 263-270.
- Colomina, M.T., Roig, J.L., Torrente, M., Vicens, P. and Domingo, J.L. (2005). Concurrent exposure to aluminum and stress during pregnancy in rats: Effects on postnatal development and behavior of the offspring. Neurotoxicol. Teratol. 27(4): 565-574.
- Confluence Engineering (2018). Personal communication. Seattle, Washington.
- Cooper, R.E. and Knowles, W.L. (1975). Loss in capacity of a large diameter water pipeline. In: Proceedings of the American Water Works Association Water Quality Technology Conference, Minneapolis, Minnesota. American Water Works Association, Denver, Colorado.
- Costello, J.J. (1984). Postprecipitation in distribution systems. J. Am. Water Works Assoc., 76(11): 46-49.
- Cui, X., Wang, B., Zong, Z., Liu, S. and Xing, W. (2012). The effects of chronic aluminum exposure on learning and memory of rats by observing the changes of ras/raf/ERK signal transduction pathway. Food Chem. Toxicol., 50(2): 315-319.
- Dahl, C., Sogaard, A.J., Tell, G.S., Flaten, T.P., Hongve, D., Omsland, T.K., Holvik, K., Meyer, H.E. and Aamodt, G. (2014). Do cadmium, lead, and aluminum in drinking water increase the risk of hip fractures? A NOREPOS study. Biol. Trace Elem. Res., 157(1): 14-23.
- Davis, C.C. and Edwards, M. (2014). Coagulation with hydrolyzing metal salts: Mechanisms and water quality impacts. Crit. Rev. Environ. Sci. Technol., 44(4): 303-347.
- Del Toral, M.A., Porter, A. and Schock, M.R. (2013). Detection and evaluation of elevated lead release from service lines: A field study. Environ. Sci. Technol., 47(16): 9300-9307.
- Dempsey, B.A. (2006). Coagulant characteristics and reactions. Chapter 2 in: Interface science in drinking water treatment: Theory and applications. G. Newcombe and D. Dixon (eds). Academic Press, London, United Kingdom. pp. 5-24.
- Dietrich, A.M. (2015). EPA secondary maximum contaminants levels: A strategy for drinking water quality and consumer acceptability. Water Research Foundation, Denver, Colorado.
- Donald, J.M., Golub, M.S., Gershwin, M.E. and Keen, C.L. (1989). Neurobehavioral effects in offspring of mice given excess aluminum in diet during gestation and lactation. Neurotoxicol. Teratol. 11(4): 345-351.
- do Nascimento, S.N., Charao, M.F., Moro, A.M., Roehrs, M., Paniz, C., Baierle, M., Brucker, N., Gioda, A., Barbosa, F., Bohrer, D., Avila, D.S. and Garcia, S.C. (2014). Evaluation of toxic metals and essential elements in children with learning disabilities from a rural area of southern Brazil. Int. J. Environ. Res. Public. Health., 11(10): 10806-10823.
- Dong, C., Cao, J., Cao, C., Han, Y., Wu, S., Wang, S. and Wang, J. (2016). Effects of fluoride and aluminum on expressions of StAR and P450scc of related steroidogenesis in guinea pigs' testis. Chemosphere, 147: 345-351.
- Driscoll, C.T. and Letterman, R.D. (1988). Chemistry and fate of Al(III) in treated drinking water. J. Environ. Eng., 114(1): 21-37.
- Driscoll, C.T. and Letterman, R.D. (1995). Factors regulating residual aluminium concentrations in treated waters. Environmetrics, 6(3): 287-305.
- Driscoll, C.T., Letterman, R.D. and Fitch, D.E. (1987). Residual aluminum in filtered water. American Water Works Association, Denver, Colorado.
- Drobyshev, E.J., Solovyev, N.D., Gorokhovskiy, B.M. and Kashuro, V.A. (2018). Accumulation patterns of sub-chronic aluminum toxicity model after gastrointestinal administration in rats. Biol. Trace Elem. Res., 185(2): 384-394.
- DWQR (2011). Investigation into the Burncrooks Incident, North-west Glasgow, 17-19 March 2011. Drinking Water Quality Regulator for Scotland. Edinburgh, Scotland. Available at: https://dwqr.scot/media/10761/dwqr-investigation-into-burncrooks-incident-march-2011.pdf.
- Edzwald, J.K. (1993). Coagulation in drinking water treatment: Particles, organics and coagulants. Water Sci. Technol., 27(11): 21-35.
- Edzwald, J.K. (2010). Dissolved air flotation and me. Water Res., 44(7): 2077-2106.
- Edzwald, J.K. (2018). Personal communication. University of Massachusetts, Potsdam, New York.
- Edzwald, J.K. and Van Benschoten, J.E. (1990). Aluminum coagulation of natural organic matter. In: Chemical water and wastewater treatment: Proceedings of the 4th Gothenburg Symposium, Madrid, Spain. pp. 341-359.
- Edzwald, J.K. and Kelley, M.B. (1998). Control of Cryptosporidium: From reservoirs to clarifiers to filters. Water Sci. Technol., 37(2): 1-8.
- Edzwald, J.K. and Kaminski, G.S. (2009). A practical method for water plants to select coagulant dosing. J. N. Engl. Water Works Assoc., 123(1): 15-31.
- Edzwald, J.K. and Haarhoff, J. (2012). Dissolved air flotation for water clarification. McGraw Hill, New York.
- Edzwald, J.K., Becker, W.C. and Wattier, K.L. (1985). Surrogate parameters for monitoring organic matter and THM precursors. J. Am. Water Works Assoc., 77(4): 122-131.
- Edzwald, J.K., Tobiason, J.E., Parento, L.M, Kelley, M.B., Kaminski, G.S., Dunn, H.J. and Gallant, P.B. (1999). Clarification and filtration performance for removal of Giardia and Cryptosporidium. In: Proceedings of the AWWA Water Quality Technology Conference, Tampa, Florida. American Water Works Association, Denver, Colorado.
- Edzwald, J.K., Tobiason, J.E., Parento, L.M., Kelley, M.B., Kaminski, G.S., Dunn, H.J. and Galant, P.B. (2000). Giardia and Cryptosporidium removals by clarification and filtration under challenge conditions. J. Am. Water Works Assoc., 92(12): 70-84.
- Edzwald, J.K., Tobiason, J.E., Udden, C.T., Kaminski, G.S., Dunn, H.J., Gallant, P.B. and Kelley, M.B. (2003). Evaluation of the effect of recycle of waste filter backwash water on plant removals of Cryptosporidium. J. Water Supply Res. Technol. Aqua, 52(4): 243-258.
- EFSA (2006). Opinion of the scientific panel on food additives, flavourings, processing aids and materials in contact with food (AFC) on a request related to the safety in the use of the activated alumina treatment for the removal of fluoride from natural mineral waters. Question No. EFSA-Q-2005-069. European Food Safety Authority. EFSA Journal, (394): 1-8. Available at: http://www.efsa.eu.int/science/afc/catindex_en.html.
- EFSA (2008). Safety of aluminium from dietary intake - scientific opinion of the panel on food additives, flavourings, processing aids and food contact materials (AFC). European Food Safety Authority. EFSA Journal, (7): 1-34.
- Eikebrokk, B. (1996). Removal of humic substances by coagulation. In: Chemical water and wastewater treatment IV. H.H. Hahn, E. Hoffmann, and H. Ødegaard (eds). Springer-Verlag, Berlin Heidelburg. pp. 173-187.
- ElBaz, F.K., Aly, H.F. and Ali, G.H. (2017). Haematococcus pluvialis modulating effect on neurotransmitters, hormones and oxidative damage-associated with Alzheimer's disease in experimental rat's model. Int. J. Pharm. Pharm. Sci., 9(2): 198-206.
- Elliott, J., Norman, G., Campbell, A. and Douglas, I. (2016). Optimizing coagulation: "Around the world in 100 jars!". Canadian National Conference on Drinking Water, Ottawa, Ontario.
- Environment and Climate Change Canada (2017). National long-term water quality monitoring data. Available at: http://donnees.ec.gc.ca/data/substances/monitor/national-long-term-water-quality-monitoring-data/
- Environment Canada and Health Canada (2010). Priority substances list assessment report. Follow-up to the state of science report 2000. Aluminum chloride, aluminum nitrate, aluminum sulphate. Ottawa. Available at: https://www.ec.gc.ca/lcpe-cepa/default.asp?lang=En&n=AA0EAAFE-1
- Erazi, H., Sansar, W., Ahboucha, S. and Gamrani, H. (2010). Aluminum affects glial system and behavior of rats. C. R. Biol., 333(1): 23-27.
- Erazi, H., Ahboucha, S. and Gamrani, H. (2011). Chronic exposure to aluminum reduces tyrosine hydroxylase expression in the substantia nigra and locomotor performance in rats. Neurosci. Lett., 487(1): 8-11.
- EU (1998). Council Directive 98/83/EC of 3 November 1998 on the quality of water intended for human consumption. Official journal of the European communities, L 330, 5/12/1998. pp.32-54.
- Exley, C. (2006). Severe cerebral congophilic angiopathy coincident with increased brain aluminium in a resident of Camelford, Cornwall, UK. J. Neurol. Neurosur. Ps., 77(7): 877-79.
- Exley, C. (2013). Aluminum in biological systems. In: Encyclopedia of metalloproteins. Aluminum in biological systems. Springer, New York. pp. 33-34.
- Exley, C., House, E., Polwart, A. and Esiri, M.M. (2012). Brain burdens of aluminum, iron, and copper and their relationships with amyloid-beta pathology in 60 human brains. J. Alzheimers Dis., 31(4): 725-730.
- Farhat, S.M., Mahboob, A. and Ahmed, T. (2017a). Cortex- and amygdala-dependent learning and nicotinic acetylcholine receptor gene expression is severely impaired in mice orally treated with AlCl3. Biol. Trace Elem. Res., 179(1): 91-101.
- Farhat, S.M., Mahboob, A., Iqbal, G. and Ahmed, T. (2017b). Aluminum-induced cholinergic deficits in different brain parts and its implications on sociability and cognitive functions in mouse. Biol. Trace Elem. Res., 177(1): 115-121.
- Flarend, R., Bin, T., Elmore, D. and Hem, S.L. (2001). A preliminary study of the dermal absorption of aluminium from antiperspirants using aluminium-26. Food Chem. Toxicol., 39(2): 163-168.
- Foley, P.D. (1980). Experience with direct filtration at Ontario's Lake Huron Treatment Plant. J. Am. Water Works Assoc., 72(3): 162-164.
- Friedman, M.J., Hill, A.S., Reiber, S.H., Valentine, R.L. and Korshin, G.V. (2010). Assessment of inorganics accumulation in drinking water system scales and sediments. Water Research Foundation, Denver, Colorado.
- Friedman, M., Hill, A., Booth, S., Hallett, M., McNeill, L., McLean, J., Sorensen, D., Hammer, T., De Haan, M., MacArthur, K. and Mitchell, K. (2016). Metals accumulation and release within the distribution system: Evaluation and mitigation. Water Research Foundation, Denver, Colorado.
- Frommell, D.M., Feld, C.M., Snoeyink, V.L., Melcher, B. and Feizoulof, C. (2004). Aluminum residual control using orthophosphate. J. Am. Water Works Assoc., 96(9): 99-109.
- Fu, Y., Jia, F.B., Wang, J., Song, M., Liu, S.M., Li, Y.F., Liu, S.Z. and Bu, Q.W. (2014). Effects of sub-chronic aluminum chloride exposure on rat ovaries. Life Sci., 100(1): 61-66.
- Fuge, R., Pearce, N.J.G. and Perkins, W.T. (1992). Unusual sources of aluminium and heavy metals in potable waters. Environ. Geochem. Health, 14(1): 15-18.
- Garzillo, E.M., Lamberti, M., Genovese, G., Pedata, P., Feola, D., Sannolo, N., Daniele, L., Trojsi, F., Monsurro, M.R. and Miraglia, N. (2014). Blood lead, manganese, and aluminum levels in a regional Italian cohort of ALS patients. J. Occup. Environ. Med., 56(10): 1062-1066.
- Geng, Y. (2005). Application of flocs analysis for coagulation optimization at the Split Lake Water Treatment Plant. M.A. Sc. Thesis, University of Manitoba, Winnipeg, Manitoba. Available at: https://mspace.lib.umanitoba.ca/xmlui/handle/1993/189
- George, S., Pandit, P., and Gupta, A.B. (2010). Residual aluminium in water defluoridated using activated alumina adsorption - Modeling and simulation studies. Water Res., 44(10): 3055-3064.
- Ghorbel, I., Chaabane, M., Elwej, A., Boudawara, O., Abdelhedi, S., Jamoussi, K., Boudawara, T. and Zeghal, N. (2016a). Expression of metallothioneins I and II related to oxidative stress in the liver of aluminium-treated rats. Arch. Physiol. Biochem., 122(4): 214-222.
- Ghorbel, I., Maktouf, S., Fendri, N., Jamoussi, K., Ellouze, C.S., Boudawara, T. and Zeghal, N. (2016b). Co-exposure to aluminum and acrylamide disturbs expression of metallothionein, proinflammatory cytokines and induces genotoxicity: Biochemical and histopathological changes in the kidney of adult rats. Environ. Toxicol., 31(9): 1044-1058.
- Ghorbel I., Amara I.B., Ktari N., Elwej A., Boudawara O., Boudawara T. and Zeghal, N. (2016c). Aluminium and acrylamide disrupt cerebellum redox states, cholinergic function and membrane-bound ATPase in adult rats and their offspring. Biol. Trace Elem. Res., 174(2): 335-346.
- Giaccio, L., Cicchella, D., Vivo, B.D., Lombardi, G. and Rosa, M.D. (2012). Does heavy metals pollution affect semen quality in men? A case of study in the metropolitan area of Naples (Italy). J. Geochem. Explor., 112: 218-225.
- Golub, M.S. and Germann, S.L. (2001). Long-term consequences of developmental exposure to aluminum in a suboptimal diet for growth and behavior of Swiss Webster mice. Neurotoxicol. Teratol., 23(4): 365-372.
- Golub, M.S., Keen, C.L. and Gershwin, M.E. (1992a). Neurodevelopmental effect of aluminum in mice: Fostering studies. Neurotoxicol. Teratol. 14(3): 177-182.
- Golub, M.S., Han, B., Keen, C.L. and Gershwin, M.E. (1992b). Effects of dietary aluminum excess and manganese deficiency on neurobehavioral endpoints in adult mice. Toxicol. Appl. Pharmacol., 112(1): 154-160.
- Golub, M.S., Han, B., Keen, C.L., Gershwin, M.E. and Tarara, R.P. (1995). Behavioral performance of Swiss Webster mice exposed to excess dietary aluminum during development or during development and as adults. Toxicol. Appl. Pharmacol., 133(1): 64-72.
- Golub, M.S., Germann, S.L., Han, B. and Keen, C.L. (2000). Lifelong feeding of a high aluminum diet to mice. Toxicology, 150(1-3): 107-117.
- Gregory, R. and Edzwald, J. K. (2011). Sedimentation and flotation. Chapter 9 in: Water quality and treatment. J. K. Edzwald (ed.). McGraw Hill, New York. pp. 9.1-9.98
- Grigg, N.S. (2010). Secondary impacts of corrosion control on distribution system and treatment plant equipment. Water Research Foundation, Denver, Colorado.
- Guo, C., Chen, P., Hsia, S., Hsu, G.W. and Liu, P. (2013). The relationship of plasma aluminum to oxidant-antioxidant and inflammation status in asthma patients. Environ. Toxicol. Pharmacol., 35(1): 30-38.
- Hackenberg, U. (1972). Chronic ingestion by rats of standard diet treated with aluminum phosphide. Toxicol. Appl. Pharmacol., 23(1): 147-158.
- Halton (2001). Investigation of material deposits in the Burlington distribution system. Regional Municipality of Halton, Oakville, Ontario.
- Hao, O.J. and Huang, C.P. (1986). Adsorption characteristics of fluoride onto hydrous alumina. J. Environ. Eng., 112(6): 1054-1069.
- Haught, R. and Fabris, M. (2002). Inorganic monitors. Chapter 6 in: Online monitoring for drinking water utilities. American Water Works Association, Denver, Colorado. pp. 133-162.
- Harrington, G. W., Chen, H-W., Harris, A. J., Xagoraraki, I., Battigelli, D. A. and Standridge, J. H. (2001). Removal of emerging waterborne pathogens. AWWA Research Foundation and American Water Works Association, Denver, Colorado.
- Health Canada (2013). Guidance on Providing Safe Drinking Water in Areas of Federal Jurisdiction. Version 2, Water and Air Quality Bureau, Healthy Environments and Consumer Safety Branch, Health Canada, Ottawa, Ontario (Catalogue No. H128-1/05-440-1E-PDF).
- Health Canada (2015). Guidelines for Canadian drinking water quality. Guideline technical document: pH. Water Quality and Health Bureau, Healthy Environments and Consumer Safety Branch, Health Canada, Ottawa, Ontario. Available at: https://www.canada.ca/content/dam/hc-sc/documents/services/publications/healthy-living/guidelines-canadian-drinking-water-quality-guideline-technical-document-ph-eng.pdf.
- Health Canada (2016). Concentration of contaminants and other chemicals in food composites: Trace elements. Canadian Total Diet Study. Health Products and Food Branch, Health Canada, Ottawa. Available at: https://www.canada.ca/en/health-canada/services/food-nutrition/food-nutrition-surveillance/canadian-total-diet-study/concentration-contaminants-other-chemicals-food-composites.html.
- Health Canada (2017). Personal communication with Anca-Maria Tugulea, Environmental and Health Sciences Research Bureau.
- Health Canada (2018a). Assessment of the impacts of temperature on the efficacy of the coagulation/flocculation process in drinking water treatment. Prepared by Y.S. Vadasarukkai for Health Canada, Water and Air Quality Bureau, Healthy Environments and Consumer Safety Branch, Ottawa, Ontario. Available upon request.
- Health Canada (2018b). Assessment of the impacts of aluminum in drinking water distribution systems. Prepared by Armview Engineering Limited for Health Canada, Water and Air Quality Bureau, Healthy Environments and Consumer Safety Branch, Ottawa, Ontario. Available upon request.
- Health Canada (2019a). Guidelines for Canadian drinking water quality. Guideline technical document: Enteric protozoa: Giardia and Cryptosporidium. Water Quality and Health Bureau, Healthy Environments and Consumer Safety Branch, Health Canada, Ottawa, Ontario. Available at https://www.canada.ca/en/health-canada/services/environmental-workplace-health/reports-publications/water-quality/enteric-protozoa-giardia-cryptosporidium.html.
- Health Canada (2019b). Guidelines for Canadian drinking water quality. Guideline technical document: Enteric viruses. Water Quality and Health Bureau, Healthy Environments and Consumer Safety Branch, Health Canada, Ottawa, Ontario. Available at: https://www.canada.ca/en/health-canada/services/publications/healthy-living/guidelines-canadian-drinking-water-quality-guideline-technical-document-enteric-viruses.html.
- Health Canada (2020a). Guidance on natural organic matter in drinking water. Water Quality and Health Bureau, Healthy Environments and Consumer Safety Branch, Health Canada, Ottawa, Ontario. Available at: https://www.canada.ca/en/health-canada/services/publications/healthy-living/guidance-natural-organic-matter-drinking-water.html.
- Health Canada (2020b). Guidance on monitoring the biological stability of drinking water in distribution systems. Document for public consultation. Water and Air Quality Bureau, Healthy Environments and Consumer Safety Branch, Health Canada, Ottawa, Ontario. Available at: https://www.canada.ca/en/health-canada/programs/consultation-guidance-biological-stability-water-distribution-systems.html.
- Health Canada (in preparation). Canadian exposure factors used in human health risk assessments. Health Canada, Ottawa, Ontario.
- Hichem, N., May, M.E., Ladhari, N., Mrabet, A. and Gharbi, R. (2014). Aluminum chloride impacts dentate gyrus structure in male adult albino Wistar rats. Tissue Cell, 46(6): 409-414.
- Hill, A. and Giani, R. (2017). Magnified manganese release after switching sources to the Sacramento River. In: Proceedings of the American Water Works Association Water Quality Technology Conference, Portland, Oregon. American Water Works Association, Denver, Colorado.
- Hill, A.S., Friedman, M.J., Reiber, S.H., Korshin, G.V. and Valentine, R.L. (2010). Behaviour of trace inorganic contaminants in drinking water distribution systems. J. Am. Water Works Assoc., 102(7): 107-118.
- Hill, A.S., Friedman, M., Hallett, M., Salo-Zieman, V., Booth, S., Hanson, A., Gupta, K., Akagi, Y., Kochiss, C., Koperski, L., Igoe, P., Kirby, L. and Harper, W. (2018). Use of flushing as a corrective action under the Revised Total Coliform Rule. Report #4653. Water Research Foundation, Denver, CO.
- Hirata-Koizumi, M., Fujii, S., Ono, A., Hirose, A., Imai, T., Ogawa, K., Ema, M. and Nishikawa, A. (2011a). Two-generation reproductive toxicity study of aluminium sulfate in rats. Food Chem. Toxicol., 31(2): 219-230.
- Hirata-Koizumi, M., Fujii, S., Ono, A., Hirose, A., Imai, T., Ogawa, K., Ema, M. and Nishikawa, A. (2011b). Evaluation of the reproductive and developmental toxicity of aluminium ammonium sulfate in a two-generation study in rats. Food Chem. Toxicol., 49(9): 1948-1959.
- Hrudey, S.E. and Hrudey, E.J. (2014). Ensuring safe drinking water: Learning from frontline experience with contamination. American Water Works Association, Denver, Colorado.
- Huang, J., Wu, J., Li, T., Song, X., Zhang, B., Zhang, P. and Zheng, X. (2011). Effect of exposure to trace elements in the soil on the prevalence of neural tube defects in a high-risk area of China. Biomed. Environ. Sci., 24(2): 94-101.
- Hudson, W.D. (1966). Studies of distribution system capacity in seven cities. J. Am. Water Works Assoc., 58(2): 157-164.
- Huck, P. and Sozański, M. (2011). Chemical basis for water technology. Chapter 3.16 in: Treatise on water science. Wilderer, P. (ed.). Elsevier, Oxford, United Kingdom. pp. 429-469.
- Huck, P.M., Emelko, M.B., Coffey, B.M., Maurizio, D. and O'Melia, C. (2001). Filter operation effects on pathogen passage. American Water Works Association Research Foundation, Denver, Colorado (Report No. 90874).
- IARC (2012). Chemical agents and related occupations. A review of human carcinogens. Occupational exposures during aluminum production. International Agency for Research on Cancer Monograph Vol 100F. IARC, Lyon.
- JECFA (2007). Evaluation of certain food additives and contaminants. Sixty-seventh report of the Joint FAO/WHO Expert Committee on Food Additives (JECFA). WHO Technical Report Series, 940, Geneva.
- JECFA (2012). Safety evaluation of certain food additives. Prepared by the Seventy-Fourth Meeting of the Joint FAO/WHO Expert Committee on Food Additives (JEFCA). WHO Food Additives Series, 65, Geneva.
- Jekel, M.R. and Heinzmann, B. (1989). Residual aluminum in drinking-water treatment. J. Water Supply Res. Technol. AQUA, 38: 281-288.
- Karakis, I., Sarov, B., Landau, D., Manor, E., Yitshak-Sade, M., Rotenberg, M., Hershkovitz, R., Grotto, I., Gurevich, E. and Novack, L. (2014). Association between prenatal exposure to metals and neonatal morbidity. J. Toxicol. Env. Health. A., 77(21): 1281-1284.
- Kazza, S. (2015). Optimisation de la filtration directe aux usines Atwater et Charles-J. des Baillets. M.A. Sc. Thesis, École Polytechnique de Montréal, Montréal, Québec. Available at: https://publications.polymtl.ca/2032/1/2015_SaraKazza.pdf
- Kettunen, R. and Keskitalo, P. (2000). Combination of membrane technology and limestone filtration to control drinking water quality. Desalination, 131(1-3): 271-283.
- Kim, E.J., Herrera, J.E., Huggins, D., Braam, J. and Koshowski, S. (2011). Effect of pH on the concentrations of lead and trace contaminants in drinking water: A combined batch, pipe loop and sentinel home study. Water Res., 45(9): 2763-2774.
- Kimura, M., Matsui, Y., Kondo, K., Ishikawa, T.B., Matsushita, T. and Shirasaki, N. (2013). Minimizing residual aluminum concentration in treated water by tailoring properties of polyaluminum coagulants. Water Res., 47(6): 2075-2084.
- Knowles, A.D., Nguyen, C.K., Edwards, M.A., Stoddart, A., McIlwain, B. and Gagnon, G.A. (2015). Role of iron and aluminum coagulant metal residuals and lead release from drinking water pipe materials. J. Environ. Sci. Health Part A Toxic Hazard. Subst. Environ. Eng., 50(4): 414-423.
- Krewski, D., Yokel, R.A., Nieboer, E., Borchelt, D., Cohen, J., Harry, J., Kacew, S., Lindsay, J., Mahfouz, A.M. and Rondeau, V. (2007). Human health risk assessment for aluminium, aluminium oxide, and aluminium hydroxide. J. Toxicol. Environ. Health, Pt. B, 10(supp1): 1-269.
- Kriewall, D., Harding, R., Maisch, E. and Schanz, L. (1996). The impact of aluminum residual on transmission main capacity. Public Works, 127(12): 28-31.
- Krishnan, K. and Carrier, R. (2008). Approaches for evaluating the relevance of multiroute exposures in establishing guideline values for drinking water contaminants. J. Environ. Sci. Health. C. Environ. Carcinog. Ecotoxicol. Rev., 26(3): 300-316.
- Krishnan, K. and Carrier, R. (2013). The use of exposure source allocation factor in the risk assessment of drinking-water contaminants. J. Toxicol. Environ. Health B Crit. Rev., 16(1): 39-51.
- Kundert, K., Meilke, L., Elford, T., Maksymetz, B. and Pernitsky, D.J. (2004). Evaluating pH adjustment to investigate seasonal changes in aluminum residuals at a large conventional water treatment plant. In: Proceedings of the American Water Works Association Water Quality Technology Conference, San Antonio, Texas. American Water Works Association, Denver, Colorado.
- Kundert, K., Emelko, M.B., Mielke, L., Elford, T. and Deng, J.F. (2014). Alberta Flood 2013-City of Calgary water treatment system resiliency. Canadian National Conference on Drinking Water, Ottawa, Ontario.
- Kvech, S. and Edwards, M. (2001). Role of aluminosilicate deposits in lead and copper corrosion. J. Am. Water Works Assoc., 93(11): 104-112.
- Laabbar, W., Elgot, A., Kissani, N. and Gamrani, H. (2014). Chronic aluminum intoxication in rat induced both serotonin changes in the dorsal raphe nucleus and alteration of glycoprotein secretion in the subcommissural organ: Immunohistochemical study. Neurosci. Lett., 577: 72-76.
- Lapointe, M. and Barbeau, B. (2019). Substituting polyacrylamide with an activated starch polymer during ballasted flocculation. J. Water Process Eng., 28: 129-134.
- Larson, T.E. and Sollo Jr., F.W. (1967). Loss in water main carrying capacity. J. Am. Water Works Assoc., 60(12): 1565-1572.
- Leroy, P., Schock, M.R., Wagner, I. and Holtshulte, H. (1996). Cement-based materials. In: Internal corrosion of water distribution systems. Second edition. American Water Works Association Research Foundation and DVGW-Technologiezentrum Wasser. pp. 313-388.
- Letterman, R.D. and Driscoll, C.T. (1988). Survey of residual aluminum in filtered water. J. Am. Water Works Assoc., 80(4): 154-158.
- Li, G., Ding, Y., Xu, H., Jin, J. and Shi, B. (2018). Characterization and release profile of (Mn, Al)-bearing deposits in drinking water distribution systems. Chemosphere, 197: 73-80.
- Li, P., Luo, W., Zhang, H., Zheng, X., Liu, C. and Ouyang, H. (2015). Effects of aluminum exposure on the bone stimulatory growth factors in rats. Biol. Trace Elem. Res., 172(1): 166-171.
- Li, X., Hu, C., Zhu, Y., Sun, H., Li, Y. and Zhang, Z. (2011a). Effects of aluminum exposure on bone mineral density, mineral, and trace elements in rats. Biol. Trace Elem. Res., 143(1): 378-385.
- Li, X., Zhang, L., Zhu, Y. and Li, Y. (2011b). Dynamic analysis of exposure to aluminum and an acidic condition on bone formation in young growing rats. Environ. Toxicol. Pharmacol., 31(2): 295-301.
- Lidsky, T.I. (2014). Is the aluminum hypothesis dead? J. Occup. Environ. Med., 56(5 Suppl): S73-9.
- Linardaki, Z.I., Orkoula, M.G., Kokkosis, A.G., Lamari, F.N. and Margarity, M. (2013). Investigation of the neuroprotective action of saffron (crocus sativus L.) in aluminum-exposed adult mice through behavioral and neurobiochemical assessment. Food Chem. Toxicol., 52: 163-170.
- Lind, P.M., Olsen, L. and Lind, L. (2012). Circulating levels of metals are related to carotid atherosclerosis in elderly. Sci. Total Environ., 416: 80-88.
- Lindquist, B., Lingstrom, P., Fandriks, L. and Birkhed, D. (2011). Influence of five neutralizing products on intra-oral pH after rinsing with simulated gastric acid. Eur. J. Oral Sci., 119(4): 301-304.
- Lipps, J.P., Chen, A.S.C. and Wang, L. (2006). Arsenic removal from drinking water by adsorptive media. US EPA demonstration project at Spring Brook Mobile Home in Wales, ME. Six-month evaluation project. Prepared for: U.S. Environmental Protection Agency, Cincinnati, Ohio. EPA/600/R-06/090.
- Liu, J., Wang, Q., Sun, X., Yang, X., Zhuang, C., Xu, F., Cao, Z. and Li, Y. (2016). The toxicity of aluminum chloride on kidney of rats. Biol. Trace Elem. Res., 173(2): 339-344.
- Llobet, J.M., Domingo, J.L., Gomez, M., Tomas, J.M. and Corbella, J. (1987). Acute toxicity studies of aluminium compounds: Antidotal efficacy of several chelating agents. Pharmacol. Toxicol., 60(4): 280-283.
- Locco, D., Waller, M., Giani, R., Nikolica, P., Hill, A. and Friedman, M. (2018). Pilot testing for optimized unidirectional flushing in the Hamilton distribution system. In: Proceedings of the American Water Works Association Annual Conference and Exposition, Las Vegas, Nevada. American Water Works Association, Denver, Colorado.
- Logsdon, G.S., Hess, A.F., Chipps, M.J. and Rachwal, A.J. (2002). Filter maintenance and operations guidance manual. Water Research Foundation, Denver, Colorado.
- Lotimer, T. (2012). The role of well integrity in reducing pathogen contamination of public groundwater supplies. Canadian Water Network Conference: Assessing pathogen fate, transport and risk in natural and engineered water treatment. Banff, Alberta, Canada, September 23-26, 2012.
- Lowermoor Incident Health Advisory Group. (1989). Water pollution at Lowermoor, North Cornwall. Report of the Lowermoor Incident Health Advisory Group. Chairman: Professor Dame Barbara Clayton. Truro: Cornwall and Isles of Scilly Health Authority.
- Lv, J., Wang, W., Krafft, T., Li, Y., Zhang, F. and Yuan, F. (2011). Effects of several environmental factors on longevity and health of the human population of Zhongxiang, Hubei, China. Biol. Trace Elem. Res., 143(2): 702-716.
- Lytle, D.A., Sorg, T.J. and Frietch, C. (2004). Accumulation of arsenic in drinking water distribution systems. Environ. Sci. Technol., 38(20): 5365-5372.
- Manitoba Sustainable Development (2017). Personal communication with Kim Philip, Office of Drinking Water.
- Martinez, C.S., Alterman, C.D.C., Pecanha, F.M., Vassallo, D.V., MelloCarpes, P.B., Miguel, M. and Wiggers, G.A. (2017a). Aluminum exposure at human dietary levels for 60 days reaches a threshold sufficient to promote memory impairment in rats. Neurotox. Res., 31(1): 20-30.
- Martinez, C.S., Escobar, A.G., Uranga-Ocio, J.A., Pecanha, F.M., Vassallo, D.V., Exley, C., Miguel, M. and Wiggers, G.A. (2017b). Aluminum exposure for 60 days at human dietary levels impairs spermatogenesis and sperm quality in rats. Reprod. Toxicol., 73: 128-141.
- Matilainen, A., Vepsäläinen, M. and Sillanpää, M. (2010). Natural organic matter removal by coagulation during drinking water treatment: A review. Adv. Colloid Interface Sci., 159(2): 189-197.
- McMillan, T.M., Dunn, G. and Colwill, S.J. (1993a). Psychological testing on schoolchildren before and after pollution of drinking water in North Cornwall. J. Child Psychol. Psychiatry, 34(8): 1449-1459.
- McMillan, T.M., Freemont, A.J., Herxheimer, A., Denton, J., Taylor, A.P., Pazianas, M., Cummin, A.R. and Eastwood, J.B. (1993b). Camelford water poisoning accident: Serial neuropsychological assessments and further observations on bone aluminium. Hum. Exp. Toxicol., 12(1): 37-42.
- McVicar, M., Bickerton, B., Chaulk, M. and Walsh, M. (2015). UV254 and streaming current monitors can improve coagulation control in challenging conditions. Opflow, 41(7): 26-28.
- Ministère du Développement durable, de l'Environnement et de la Lutte contre les changements climatiques (2017). Personal communication with Caroline Robert, Direction de l'eau potable et des eaux souterraines.
- Mirza, A., King, A., Troakes, C. and Exley, C. (2017). Aluminium in brain tissue in familial Alzheimer's disease. J. Trace Elem. Med. Biol., 40: 30-36.
- Mlynska, A. and Zielina, M. (2017). The influence of prefabricated pipe cement coatings and those made during pipe renovation on drinking water quality. In: E3S Web of Conferences. 17. 00061. 10.1051/e3sconf/20171700061.
- Morfesis, A., Jacobson, A.M., Frollini, R., Helgeson, M., Billica, J. and Gertig, K.R. (2009). Role of zeta (ζ) potential in the optimization of water treatment facility operations. Ind. Eng. Chem. Res., 48: 2305-2308.
- Munk, L. and Faure, G. (2004). Effects of pH fluctuations on potentially toxic metals in the water and sediment of the Dillon Reservoir, Summit County, Colorado. Appl. Geochem., 19(7): 1065-1074.
- Muselin, F., Cristina, R.T., Igna, V., Dumitrescu, E., Brezovan, D. and Trif, A. (2016). The consequences of aluminium intake on reproductive function in male rats: A three-generation study. Turk. J. Med. Sci., 46(4): 1240-1248.
- Muylwyk, Q. and MacDonald, J. (2001). Aluminum deposits in the distribution system: What can you do? In: Proceedings of the American Water Works Association Water Quality Technology Conference, Nashville, Tennessee. American Water Works Association, Denver, Colorado.
- MWH. (2012). Water treatment principles and design. 3rd edition. John Wiley & Sons, New York, New York.
- New Brunswick Department of Environment and Local Government (2018). Personal communication with Kevin Gould, Healthy Environment Branch.
- Newfoundland and Labrador Department of Municipal Affairs and Environment (2017). Personal communication with Haseen Khan, Water Resources Management Division.
- New Zealand Ministry of Health (2008). Drinking-water standards for New Zealand 2005 (Revised 2008). Ministry of Health, Wellington.
- NHMRC and NRMMC (2011). Australian drinking water guidelines. Paper 6. National Water Quality Management Strategy. National Health and Medical Research Council, National Resource Management Ministerial Council, Commonwealth of Australia, Canberra, Version 3.4. Updated October 2017. Available at: https://www.nhmrc.gov.au/_files_nhmrc/file/publications/nhmrc_adwg_6_version_3.4_final.pdf
- Nicolescu, R., Petcu, C., Cordeanu, A., Fabritius, K., Schlumpf, M., Krebs, R., Kramer, U. and Winneke, G. (2010). Environmental exposure to lead, but not other neurotoxic metals, relates to core elements of ADHD in Romanian children: Performance and questionnaire data. Environ. Res., 110(5): 476-483.
- Nova Scotia Environment (2018). Personal communication with Angelina Polegato, Drinking Water Management Unit.
- NRC (2006). Drinking water distribution systems: Assessing and reducing risks. National Research Council. The National Academies Press, Washington, DC.
- NRCan (2018). Aluminum facts, Natural Resources Canada. Last Updated: April 20, 2018. Government of Canada, Ottawa. Available at: http://www.nrcan.gc.ca/mining-materials/facts/aluminum/20510
- NSCFS (2013). Risk Assessment of the exposure to aluminum through food and the use of cosmetic products in the Norwegian population. Opinion of the Panel on Food Additives, Flavourings, Processing Aids, Materials in Contact with Food and Cosmetics and of the Panel on Contaminants of the Norwegian Scientific Committee for Food Safety. Doc. No. 11-504_final. Available at: https://vkm.no/download/18.175083d415c86c573b59c179/1501678206406/a729a67e65.pdf
- NSF (2018). Aluminum contaminant concentrations in water treatment chemicals. Prepared for Health Canada, Ottawa, Ontario.
- NSF/ANSI/CAN (2020). NSF International/American National Standards Institute/National Standard of Canada Standard 60: Drinking water treatment chemicals: Health effects. NSF International, Ann Arbor, Michigan.
- NSF/ANSI/CAN (2019a). NSF International/American National Standards Institute/National Standard of Canada Standard 600: Health effects evaluation and criteria for chemicals in drinking water. NSF International, Ann Arbor, Michigan.
- NSF/ANSI/CAN (2019b). NSF International/American National Standards Institute/National Standard of Canada Standard 61: Drinking water system components: Health effects. NSF International, Ann Arbor, Michigan.
- O'Melia, C.R. (2006). Fundamentals of particle stability. Chapter 18 in: Interface science in drinking water treatment: theory and applications. G. Newcombe and D. Dixon (eds). Academic Press, London, United Kingdom. pp. 317-362.
- Ondreicka, R., Ginter, E. and Kortus, J. (1966). Chronic toxicity of aluminium in rats and mice and its effects on phosphorus metabolism. Br. J. Ind. Med., 23(4): 305-312.
- Oneda, S., Takasaki, T., Kuriwaki, K., Ohi, Y., Umekita, Y., Hatanaka, S., Fujiyoshi, T., Yoshida, A. and Yoshida, H. (1994). Chronic toxicity and tumorigenicity study of aluminum potassium sulfate in B6C3F1 mice. In Vivo, 8(3): 271-278.
- Ontario Ministry of the Environment and Climate Change (2017). Selected water quality results for treated and distribution water from the drinking water surveillance program in Ontario treatment plants (2013-2017). Available at: https://www.ontario.ca/data/drinking-water-surveillance-program.
- Ontario Ministry of Agriculture, Food and Rural Affairs (1997). Best management practices: Water wells. Available at: http://www.omafra.gov.on.ca/english/environment/bmp/well.htm.
- Oshima, E., Ishihara, T., Yokota, O., Nakashima-Yasuda, H., Nagao, S., Ikeda, C., Naohara, J., Terada, S. and Uchitomi, Y. (2013). Accelerated tau aggregation, apoptosis and neurological dysfunction caused by chronic oral administration of aluminum in a mouse model of tauopathies. Brain Pathol., 23(6): 633-644.
- Oteiza, P.I., Keen, C.L., Han, B. and Golub, M.S. (1993). Aluminum accumulation and neurotoxicity in Swiss-Webster mice after long-term dietary exposure to aluminum and citrate. Metabolism, 42(10): 1296-1300.
- Panhwar, A.H., Kazi, T.G., Afridi, H.I., Arain, S.A., Arain, M.S., Brahaman, K.D., Naeemullah and Arain, S.S. (2016). Correlation of cadmium and aluminum in blood samples of kidney disorder patients with drinking water and tobacco smoking: Related health risk. Environ. Geochem. Health, 38(1): 265-274.
- Pernitsky, D.J. (2003). Coagulation 101. In: Proceedings of the Technology Transfer Conference, University of Calgary, Alberta, Canada.
- Pernitsky, D. (2019). Coagulation 101. In: Proceedings of the American Water Works Association Annual Conference and Exposition, Denver, Colorado. American Water Works Association, Denver, Colorado.
- Pernitsky, D.J. and Edzwald, J.K. (2003). Solubility of polyaluminium coagulants. J. Water Supply Res. Technol. Aqua, 52(6): 395-406.
- Pernitsky, D.J. and Edzwald, J.K. (2006). Selection of alum and polyaluminum coagulants: Principles and applications. J. Water Supply Res. Technol. AQUA, 55(2): 121-141.
- Pineau, A., Guillard, O., Favreau, F., Marrauld, A. and Fauconneau, B. (2012). In vitro study of percutaneous absorption of aluminum from antiperspirants through human skin in the Franz diffusion cell. J. Inorg. Biochem., 110: 21-26.
- Plummer, J.D., Edzwald, J.K. and Kelley, M.B. (1995). Removing Cryptosporidium by dissolved air flotation. J. Am. Water Works Assoc., 87(9):85-95.
- Poirier, J., Semple, H., Davies, J., Lapointe, R., Dziwenka, M., Hiltz, M. and Mujibi, D. (2011). Double-blind, vehicle-controlled randomized twelve-month neurodevelopmental toxicity study of common aluminum salts in the rat. Neuroscience, 193: 338-362.
- Priest, N.D. (2004). The biological behaviour and bioavailability of aluminium in man, with special reference to studies employing aluminium-26 as a tracer: Review and study update. J. Environ. Monit., 6(5): 375-403.
- Priest, N., Newton, D., Day, J., Talbot, R. and Warner, A. (1995). Human metabolism of aluminium-26 and gallium-67 injected as citrates. Hum. Exp. Toxicol., 14(3): 287-293.
- Rao, S.S. and Adlard, P.A. (2018). Untangling tau and iron: Exploring the interaction between iron and tau in neurodegeneration. Front. Mol. Neurosci., 11: 276.
- Randall, S. (2020). Personal communication. NSF International, Ann Arbor, Michigan.
- Reijnen, G.K., Kostense, A., Verdouw, J. and Hiemstra, P. (1991). Aluminum in groundwater, origin, sampling, analysis and removal. In: Proceedings of the International Water Supply Association, Copenhagen, Denmark. International Water Supply Association, The Netherlands.
- Rondeau, V., Jacqmin-Gadda, H., Commenges, D., Helmer, C. and Dartigues, J.F. (2009). Aluminum and silica in drinking water and the risk of Alzheimer's disease or cognitive decline: Findings from 15-year follow-up of the PAQUID cohort. Am. J. Epidemiol., 169(4): 489-496.
- Rui, D. and Yongjian, Y. (2010). Aluminum chloride induced oxidative damage on cells derived from hippocampus and cortex of ICR mice. Brain Res., 1324: 96-102.
- Rusina, R., Matej, R., Kasparova, L., Kukal, J. and Urban, P. (2011). Higher aluminum concentration in Alzheimer's disease after box-cox data transformation. Neurotox. Res., 20(4): 329-333.
- Saskatchewan Water Security Agency (2017). Personal communication with Sam Ferris, Environmental and Municipal Management Services Division.
- Schock, M.R. (2005). Distribution systems as reservoirs and reactors for inorganic contaminants. Chapter 6 in: Distribution system water quality challenges in the 21st century: A strategic guide. American Water Works Association, Denver, Colorado. pp. 105-140.
- Schock, M.R. and Holm, T.R. (2003). Are we monitoring in the right places for inorganics and radionuclides? J. N. Engl. Water Works Assoc., 117(2): 102-116.
- Schreurs, B.G. and Sparks, D.L. (2016). Dietary high cholesterol and trace metals in the drinking water increase levels of ABCA1 in the rabbit hippocampus and temporal cortex. J. Alzheimers Dis., 49(1): 201-209.
- Schroeder, H.A. and Mitchener, M. (1975a). Life-term effects of mercury, methyl, mercury, and nine other trace metals on mice. J. Nutr., 105(4): 452-458.
- Schroeder, H.A. and Mitchener, M. (1975b). Life-term studies in rats: Effects of aluminum, barium, beryllium and tungsten. J. Nutr., 105(4): 421-427.
- Sharp, E. (2015). Using online zeta potential measurements for coagulation control: A first for the UK water industry. In: Proceedings of the IWA Specialist Conference on Natural Organic Matter in Drinking Water, Malmo, Sweden.
- Sharp, E.L., Parsons, S.A. and Jefferson, B. (2006). Seasonal variations in natural organic matter and its impact on coagulation in water treatment. Sci. Total Environ., 363(1-3): 183-194.
- She, Y., Zhao, H., Zhu, Y., Han, Y., Xia, S., Bai, C., Zhang, J. and Li, Y. (2015). Aluminum trichloride disorders bile acid secretion and induces hepatocyte apoptosis in rats. Cell Biochem. Biophys., 71(3): 1569-1577.
- Shin, J.Y., Spinette, R.F. and O'Melia, C.R. (2008). Stoichiometry of coagulation revisited. Environ. Sci. Technol., 42(7): 2582-2589.
- Sillanpää, M. and Matilainen, A. (2015). NOM removal by coagulation. Chapter 3 in: Natural organic matter in water: Characterization and treatment methods. M. Sillanpää (ed.). IWA Publishing, Oxford, United Kingdom. pp 55-80.
- Sillanpää, M., Ncibi, M.C., Matilainen, A., and Vepsäläinen, M. (2018). Removal of natural organic matter in drinking water treatment by coagulation: A comprehensive review. Chemosphere, 190: 54-71.
- Simms, J. and Azizian, F. (1997). Pilot-plant trials on the removal of arsenic from potable water using activated alumina. In: Proceedings of the American Water Works Association Water Quality Technology Conference, Denver, Colorado. American Water Works Association, Denver, Colorado.
- Singla, N. and Dhawan, D.K. (2017). Zinc improves cognitive and neuronal dysfunction during aluminium-induced neurodegeneration. Mol. Neurobiol., 54(1): 406-422.
- Skalnaya, M.G., Tinkov, A.A., Demidov, V.A., Serebryansky, E.P., Nikonorov, A.A. and Skalny, A.V. (2014). Hair toxic element content in adult men and women in relation to body mass index. Biol. Trace Elem. Res., 161(1): 13-19.
- Smeets, P.W.M.H., Medema, G.J. and Van Dijk, J.C. (2009). The Dutch secret: How to provide safe drinking water without chlorine in The Netherlands. Drink. Water Eng. Sci., 2(1): 1-14.
- Snoeyink, V.L., Schock, M.R., Sarin, P., Wang, L., Chen, A.S-C. and Harmon, S.M. (2003). Aluminium-containing scales in water distribution systems: Prevalence and composition. J. Water Supply Res. Technol. AQUA, 52(7): 455-474.
- Srinivasan, P.T., Viraraghavan, T. and Bergman, J. (1999). Factors influencing residual aluminium levels at the Buffalo Pound Water Treatment Plant, Saskatchewan, Canada. J. Water Supply Res. Technol. AQUA, 48(4): 167-175.
- Statistics Canada (2013). Survey of drinking water plants 2011. Minister of Industry, 16-403-X, Ottawa.
- Stauber, J., Florence, T., Davies, C., Adams, M. and Buchanan, S. (1999). Bioavailability of Al in alum-treated drinking water. J. Am. Water Works Assoc., 91(11): 84-93.
- Strozyk, D., Launer, L.J., Adlard, P.A., Cherny, R.A., Tsatsanis, A., Volitakis, I., Blennow, K., Petrovitch, H., White, L.R. and Bush, A.I. (2009). Zinc and copper modulate Alzheimer Abeta levels in human cerebrospinal fluid. Neurobiol. Aging, 30(7): 1069-1077.
- Sun, H., Hu, C., Jia, L., Zhu, Y., Zhao, H., Shao, B., Wang, N., Zhang, Z. and Li, Y. (2011). Effects of aluminum exposure on serum sex hormones and androgen receptor expression in male rats. Biol. Trace Elem. Res., 144(1): 1050-1058.
- Sun, X., Cao, Z., Zhang, Q., Liu, S., Xu, F., Che, J., Zhu, Y., Li, Y., Pan, C. and Liang, W. (2015). Aluminum trichloride impairs bone and downregulates Wnt/β-catenin signaling pathway in young growing rats. Food Chem. Toxicol., 86: 154-162.
- Sun, X., Liu, J., Zhuang, C., Yang, X., Han, Y., Shao, B., Song, M., Li, Y. and Zhu, Y. (2016). Aluminum trichloride induces bone impairment through TGF-β1/Smad signaling pathway. Toxicology, 371: 49-57.
- Sun, X., Wang, H., Huang, W., Yu, H., Shen, T., Song, M., Han, Y., Li, Y. and Zhu, Y. (2017). Inhibition of bone formation in rats by aluminum exposure via Wnt/β-catenin pathway. Chemosphere, 176: 1-7.
- Talbot, R.J., Newton, D., Priest, N.D., Austin, J.G. and Day, J.P. (1995). Inter-subject variability in the metabolism of aluminium following intravenous injection as citrate. Hum. Exp. Toxicol., 14(7): 595-599.
- Tamburo, E., Varrica, D., Dongarra, G. and Grimaldi, L.M. (2015). Trace elements in scalp hair samples from patients with relapsing-remitting multiple sclerosis. PLoS One, 10(4): e0122142.
- Trueman, B.F. and Gagnon, G.A. (2016). A new analytical approach to understanding nanoscale lead-iron interactions in drinking water distribution systems. J. Hazard. Mater., 311: 151-157.
- Turkez, H., Yousef, M.I. and Geyikoglu, F. (2010). Propolis prevents aluminium-induced genetic and hepatic damages in rat liver. Food Chem. Toxicol., 48(10): 2741-2746.
- UK Committee on Toxicology. (2013). Subgroup report on the Lowermoor water pollution incident. Available at: https://cot.food.gov.uk/sites/default/files/cot/lwpiapp811.pdf
- US EPA (1994a). Method 200.7 revision 4.4. Determination of metals and trace elements in water and wastes by inductively coupled plasma-atomic emission spectrometry. Environmental Monitoring Systems Laboratories. Office of Research and Development, United States Environmental Protection Agency, Cincinnati, Ohio.
- US EPA (1994b). Method 200.8 revision 5.4. Determination of trace elements in waters and wastes by inductively coupled plasma - mass spectrometry. Environmental Monitoring Systems Laboratories, Office of Research and Development, United States Environmental Protection Agency, Cincinnati, Ohio.
- US EPA (1994c). Method 200.9. revision 4.2. Determination of trace elements by stabilized temperature graphite furnace atomic adsorption. Environmental Monitoring Systems Laboratories, Office of Research and Development, United States Environmental Protection Agency, Cincinnati, Ohio.
- US EPA (2002). Distribution system issue paper - Permeation and leaching. Prepared by AWWA with assistance from Economic and Engineering Services, Inc. for US EPA, Office of Water, Office of Ground Water and Drinking Water.
- US EPA (2003). Method 200.5 revision 4.2. Determination of trace elements in drinking water by axially viewed inductively coupled plasma - atomic emission spectrometry. EPA 600-R-06-115. National Exposure Research Laboratory, Office of Research and Development, United States Environmental Protection Agency, Cincinnati, Ohio.
- Valade, M.T., Becker, W.C. and Edzwald, J.K. (2009). Treatment selection guidelines for particle and NOM removal. J. Water Supply Res. Technol. Aqua, 58(6): 424-432.
- Valigore, J.M., Chen, A.S.C. and Wang, L. (2007). Arsenic removal from drinking water by adsorptive media. US EPA demonstration project at Valley Vista, AZ. Final performance evaluation report. Prepared for: U.S. Environmental Protection Agency, Cincinnati, Ohio. EPA/600/R-07/133.
- Van Benschoten, J.E. and Edzwald, J.K. (1990a). Chemical aspects of coagulation using aluminum salts-II. Coagulation of fulvic acid using alum and polyaluminum chloride. Water Res., 24(12): 1527-1535.
- Van Benschoten, J.E. and Edzwald, J.K. (1990b). Measuring aluminum during water treatment. Methodology and application. J. Am. Water Works Assoc., 82(5): 71-78.
- Van Benschoten, J.E., Edzwald, J.K. and Rahman, M.A. (1992). Effects of temperature and pH on residual aluminium for alum and polyaluminium coagulants. Water Supply, 10(4): 49-54.
- Vandenplas, Y., Castrellon, P.G., Rivas, R., Gutierrez, C.J., Garcia, L.D., Jimenez, J.E., Anzo, A., Hegar, B. and Alarcon, P. (2014). Safety of soya-based infant formulas in children. Br. J. Nutr., 111(8): 1340-1360.
- Vanloot, P., Boudenne, J-L., Brach-Papa, C., Sergent, M. and Coulomb, B. (2007). An experimental design to optimize the flow extraction parameters for the selective removal of Fe(III) and Al(III) in aqueous samples using salicylic acid grafted on Amberlite XAD-4 and final determination by GF-AAS. J. Hazard. Mater., 147(1-2): 463-470.
- Virk, S.A. and Eslick, G.D. (2015). Aluminum levels in brain, serum, and cerebrospinal fluid are higher in Alzheimer's disease cases than in controls: A series of meta-analyses. J. Alzheimers Dis., 47(3): 629-638.
- Vucetic-Arsic, S., Radonjic, N.V., Jovanovic, M., Selakovic, V., Nikolic, T., Velimirovic, M., Stojkovic, T., Milovanovic, A., Milovanovic, J. and Petronijevic, N. (2013). Oxidative stress precedes mitochondrial dysfunction in gerbil brain after aluminum ingestion. Environ. Toxicol. Pharmacol., 36(3): 1242-1252.
- Walton, J.R. (2010). Evidence for participation of aluminum in neurofibrillary tangle formation and growth in Alzheimer's disease. J. Alzheimers Dis., 22(1): 65-72.
- Walton, J.R. (2014). Chronic aluminum intake causes Alzheimer's disease: Applying Sir Austin Bradford Hill's causality criteria. J. Alzheimers Dis., 40(4): 765-838.
- Wang, L., Chen, A. and Fields, K. (2000). Arsenic removal from drinking water by ion exchange and activated alumina plants. Prepared for: U. S. Environmental Protection Agency, Cincinnati, Ohio. EPA/600/R-00/088.
- Wang, B., Xing, W., Zhao, Y. and Deng, X. (2010). Effects of chronic aluminum exposure on memory through multiple signal transduction pathways. Environ. Toxicol. Pharmacol., 29(3): 308-313.
- Wang, N., She, Y., Zhu, Y., Zhao, H., Shao, B., Sun, H., Hu, C. and Li, Y. (2012). Effects of subchronic aluminum exposure on the reproductive function in female rats. Biol. Trace Elem. Res., 145(3): 382-387.
- Wang, W., Zhang, X., Wang, H., Wang, X., Zhou, L., Liu, R. and Liang, Y. (2012a). Laboratory study on the adsorption of Mn2+ on suspended and deposited amorphous Al(OH)3 in drinking water distribution systems. Water Res., 46(13): 4063-4070.
- Wang, W., Li, H., Ding, Z., Wang, X. and Liu, R. (2012b). Effects of humic acid on residual Al control in drinking water treatment plants with orthophosphate addition. Front. Environ. Sci. Eng. China, 6(4): 470-476.
- Wang, Z., Wei, X., Yang, J., Suo, J., Chen, J., Liu, X. and Zhao, X. (2016). Chronic exposure to aluminum and risk of Alzheimer's disease: A meta-analysis. Neurosci. Lett., 610: 200-206.
- Wasana, H.M., Perera, G.D., De Gunawardena, P.S. and Bandara, J. (2015). The impact of aluminum, fluoride, and aluminum-fluoride complexes in drinking water on chronic kidney disease. Environ. Sci. Pollut. Res. Int., 22(14): 11001-11009.
- Wasserstrom, L.W., Miller, S.A., Triantafyllidou, S., Desantis, M.K. and Schock, M.R. (2017). Scale formation under blended phosphate treatment for a utility with lead pipes. J. Am. Water Works Assoc., 109(11): E464-E478.
- Westerhoff, P.K., Benn, T.M., Chen, A.S.C., Wang, L. and Cumming, L.J. (2008). Assessing arsenic removal by metal (hydro)oxide adsorptive media using rapid small scale column tests. Prepared for: U.S. Environmental Protection Agency, Cincinnati, Ohio. EPA/600/R-08/051.
- WHO (2010). Aluminium in drinking-water. Background document for development of WHO guidelines for drinking-water quality. World Health Organization, WHO/HSE/WSH/10.01/13, Geneva.
- WHO (2011a). Guidelines for drinking-water quality. Fourth edition. World Health Organization, Geneva, Switzerland. Available at: http://www.who.int/water_sanitation_health/publications/2011/dwq_guidelines/en/
- WHO (2011b). Water safety in buildings. WHO Press. Geneva, Switzerland. Available at: https://www.who.int/water_sanitation_health/publications/2011/9789241548106/en/
- WHO (2012). Water safety planning for small community water supplies. World Health Organization, Geneva, Switzerland. Available at: http://www.who.int/water_sanitation_health/publications/small-comm-water_supplies/en/
- Willhite, C.C., Karyakina, N.A., Yokel, R.A., Yenugadhati, N., Wisniewski, T.M., Arnold, I.M., Momoli, F. and Krewski, D. (2014). Systematic review of potential health risks posed by pharmaceutical, occupational and consumer exposures to metallic and nanoscale aluminum, aluminum oxides, aluminum hydroxide and its soluble salts. Crit. Rev. Toxicol., 44 (Suppl. 4): 1-80.
- Xu, L., Zhang, W., Liu, X., Zhang, C., Wang, P. and Zhao, X. (2018). Circulatory levels of toxic metals (aluminum, cadmium, mercury, lead) in patients with Alzheimer's disease: A quantitative meta-analysis and systematic review. J. Alzheimers Dis., 62(1): 361-372.
- Yokel, R.A. and McNamara, P.J. (1985). Aluminum bioavailability and disposition in adult and immature rabbits. Toxicol. Appl. Pharmacol., 77(2): 344-352.
- Yu, L., Zhai, Q., Liu, X., Wang, G., Zhang, Q., Zhao, J., Narbad, A., Zhang, H., Tian, F. and Chen, W. (2016). Lactobacillus plantarum CCFM639 alleviates aluminium toxicity. Appl. Microbiol. Biotechnol., 100(4): 1891-1900.
- Yukon Health and Social Services. (2017). Personal communication with Pat Brooks, Drinking Water Program Coordinator.
- Zhang, C., Li, Y., Wang, C., Lv, R. and Song, T. (2013a). Extremely low-frequency magnetic exposure appears to have no effect on pathogenesis of Alzheimer's disease in aluminum-overloaded rat. PLoS One, 8(8): e71087.
- Zhang, L., Jin, C., Liu, Q., Lu, X., Wu, S., Yang, J., Du, Y., Zheng, L. and Cai, Y. (2013b). Effects of subchronic aluminum exposure on spatial memory, ultrastructure and L-LTP of hippocampus in rats. J. Toxicol. Sci., 38(2): 255-268.
- Zhang, L., Jin, C., Lu, X., Yang, J., Wu, S., Liu, Q., Chen, R., Bai, C., Zhang, D., Zheng, L., Du, Y. and Cai, Y. (2014). Aluminium chloride impairs long-term memory and downregulates cAMP-PKA-CREB signalling in rats. Toxicology, 323(Suppl. C): 95-108.
- Zhou, Y, and Yokel, R.A. (2005). The chemical species of aluminum influences its paracellular flux across and uptake into Caco-2 cells, a model of gastrointestinal absorption. Tox. Sci. 87(1), 15-26.
- Zhu, Y., Han, Y., Zhao, H., Li, J., Hu, C., Li, Y. and Zhang, Z. (2013). Suppressive effect of accumulated aluminum trichloride on the hepatic microsomal cytochrome P450 enzyme system in rats. Food Chem. Toxicol., 51(Suppl. C): 210-214.
- Zhu, Y.Z., Sun, H., Fu, Y., Wang, J., Song, M., Li, M., Li, Y.F. and Miao, L.G. (2014). Effects of sub-chronic aluminum chloride on spermatogenesis and testicular enzymatic activity in male rats. Life Sci., 102(1): 36-40.
- Zielina, M., Mlynska, A. and Zaba, T. (2015). Experimental research on deterioration of drinking water quality after cement mortar pipe lining. Technical Transactions: Civil Engineering, 4-B: 145-152.
Appendix A: List of abbreviations
- Aβ
- Beta-amyloid
- AChE
- Acetylcholinesterase
- AD
- Alzheimer's disease
- ANSI
- American National Standards Institute
- APHA
- American Public Health Association
- ATSDR
- Agency for Toxic Substances and Disease Registry
- AWWA
- American Water Works Association
- bw
- body weight
- CAN
- National Standard of Canada
- CCME
- Canadian Council of Ministers of the Environment
- DWQR
- Drinking Water Quality Regulator (for Scotland)
- EFSA
- European Food Safety Authority
- GLP
- Good laboratory practice
- GSH
- Glutathione
- HBV
- Health-based value
- JECFA
- Joint Food and Agriculture Organization of the United Nations/World Health Organization Expert Committee on Food Additives
- LD50
- median lethal dose
- LOAEL
- Lowest-observed-adverse-effect level
- MAC
- Maximum acceptable concentration
- MDA
- Malondialdehyde
- MDL
- Method detection limit
- NHMRC
- National Health and Medical Research Council (Australia)
- NOAEL
- No-observed-adverse-effect level
- NOM
- Natural organic matter
- NRMCC
- Natural Resources Management Ministerial Council (Australia)
- NSF
- NSF International
- NTU
- Nephelometric turbidity unit
- OECD
- Organization for Economic Co-operation and Development
- OG
- Operational guidance
- PACl
- Polyaluminum chloride
- PM10
- Particulate matter, 10 micrometres in diameter or less
- PTs
- Provinces and territories
- PTWI
- Provisional Tolerable Weekly Intake
- QA/QC
- Quality assurance and quality control
- SM
- Standard Method
- SOD
- Superoxide dismutase
- SUVA
- Specific ultraviolet absorbance
- TDI
- Tolerable daily intake
- US EPA
- United States Environmental Protection Agency
- WHO
- World Health Organization
Appendix B: Canadian water quality data
Water Type | Summer (μg/L)Table B-1 footnote a,Table B-1 footnote b | Winter (μg/L)Table B-1 footnote a,Table B-1 footnote b | ||||||||
---|---|---|---|---|---|---|---|---|---|---|
Detects/ samples |
Median | Mean | 90th | Max | Detects/ samples |
Median | Mean | 90th | Max | |
Well-raw | 7/17 | 8 | 10 | 17 | 17 | 6/9 | 8 | 28 | 70 | 130 |
Well-treated | 9/16 | 9 | 12 | 24 | 32 | 7/9 | 6 | 12 | 26 | 36 |
Well-distribution | 6/17 | 19 | 17 | 27 | 31 | 6/9 | 15 | 16 | 24 | 31 |
Lake-raw | 16/16 | 27 | 59 | 146 | 310 | 10/11 | 16 | 39 | 72 | 230 |
Lake-treated | 16/16 | 21 | 34 | 71 | 120 | 10/11 | 14 | 52 | 114 | 280 |
Lake-distribution | 21/21 | 16 | 56 | 130 | 330 | 8/8 | 23 | 43 | 99 | 140 |
River-raw | 22/22 | 175 | 462 | 1 172 | 2 600 | 11/11 | 91 | 357 | 370 | 2 800 |
River-treated | 22/22 | 35 | 89 | 220 | 390 | 9/11 | 53 | 74 | 122 | 270 |
River-distribution | 26/26 | 34 | 68 | 155 | 330 | 9/10 | 43 | 55 | 95 | 210 |
Source: Health Canada, 2017a. |
Region | River basin | No. of samples | No. of detectsTable B-2 footnote a,Table B-2 footnote b | Median (μg/L) |
Mean (μg/L) |
90th percentile (μg/L) |
Maximum (μg/L) |
---|---|---|---|---|---|---|---|
East | Maritime Coast | 583 | 583 | 168 | 337 | 335 | 84 800 |
Newfoundland-Labrador | 1 127 | 1 126 | 82 | 128 | 216 | 4 120 | |
North Shore-Gaspé | 42 | 42 | 113 | 140 | 166 | 887 | |
Saint John-St. Croix | 89 | 88 | 35 | 72 | 153 | 634 | |
Central | Winnipeg | 53 | 53 | 166 | 173 | 248 | 347 |
Prairie | Assiniboine-Red | 829 | 827 | 320 | 875 | 2 348 | 16 100 |
Churchill | 292 | 280 | 38 | 97 | 235 | 1 880 | |
Lower Saskatchewan-Nelson | 394 | 394 | 161 | 362 | 960 | 3 120 | |
Missouri | 94 | 94 | 280 | 1 052 | 1 744 | 22 800 | |
North Saskatchewan | 491 | 491 | 105 | 525 | 1 060 | 19 300 | |
South Saskatchewan | 750 | 748 | 66 | 925 | 1 440 | 58 500 | |
Pacific | Columbia | 4 418 | 4 395 | 25 | 138 | 348 | 9 850 |
Fraser | 3 689 | 3 689 | 167 | 617 | 1 580 | 24 800 | |
Okanagan-Similkameen | 1 153 | 1 152 | 41 | 287 | 542 | 21 200 | |
Pacific Coastal | 2 789 | 2 789 | 123 | 693 | 1 762 | 25 900 | |
Peace-Athabasca | 393 | 393 | 121 | 776 | 1 896 | 21 000 | |
Arctic | Arctic Coast | 136 | 136 | 392 | 2 357 | 6 275 | 26 600 |
Keewatin-Southern Baffin Island | 39 | 39 | 11 | 13 | 24 | 39 | |
Lower Mackenzie | 919 | 916 | 73 | 577 | 1 510 | 12 800 | |
Yukon | 642 | 632 | 42 | 165 | 454 | 3 080 | |
Source: Environment Canada, 2017. |
Footnotes
- Footnote 1
-
Locational running annual average means the average concentration for samples collected at a certain location and at the specified frequency for the previous 12 months.
Page details
- Date modified: