Guidance on Monitoring the Biological Stability of Drinking Water in Distribution Systems

Download the alternative format
(PDF format, 1.2 MB, 41 pages)
Organization: Health Canada
Published: 2022-02-25
Background on guidance documents
Health Canada works with the provinces, territories and federal agencies to establish the Guidelines for Canadian Drinking Water Quality. Over the years, new methodologies and approaches have led Health Canada, in collaboration with the Federal-Provincial-Territorial Committee on Drinking Water, to develop a new type of document, guidance documents, to provide advice and guidance on issues related to drinking water quality for parameters that do not require a formal Guideline for Canadian Drinking Water Quality.
Guidance documents are developed to provide operational or management guidance related to specific drinking water-related issues (e.g., boil water advisories), to make health risk assessment information available when a guideline is not deemed necessary.
Guidelines are established under the Guidelines for Canadian Drinking Water Quality specifically for contaminants that meet all of the following criteria:
- exposure to the contaminant could lead to adverse health effects;
- the contaminant is frequently detected or could be expected to be found in a large number of drinking water supplies throughout Canada; and
- the contaminant is detected, or could be expected to be detected, at a level that is of possible health significance.
If a contaminant of interest does not meet all these criteria, Health Canada, in collaboration with the Federal-Provincial Territorial Committee on Drinking Water, may choose not to develop a Guideline Technical Document. In that case, a guidance document may be developed.
Guidance documents undergo a similar process as Guideline Technical Documents, including public consultations through the Health Canada Web site. They are offered as information for drinking water authorities and to help provide guidance in spill or other emergency situations.
Part A of this document provides the guidance for monitoring biological stability of drinking water in distribution systems; Part B provides the scientific and technical information to support this guidance; and Part C provides the references.
Executive summary
The drinking water distribution system is the last protective barrier before the consumer's tap. A well-maintained and operated distribution system is therefore a critical component of providing safe drinking water. In order to maintain water quality in the distribution system, it is essential to understand when changes occur. This understanding is achieved through the use of monitoring aimed at assessing the biological stability of water in the distribution system.
Health Canada completed its review of biological stability of drinking water in distribution systems. This guidance document was prepared in collaboration with the Federal-Provincial-Territorial Committee on Drinking Water and describes the significance of biological stability in drinking water distribution systems, monitoring approaches and best practices designed to ensure safe drinking water.
Assessment
Distribution systems represent a complex and dynamic environment, where numerous physical, chemical and biological interactions and reactions capable of significantly impacting water quality can occur. As a consequence, illness, including waterborne outbreaks, has been linked to degradation in distribution system water quality. Despite this, drinking water distribution systems, and the changes in biological stability within them, are generally not characterized or well-understood. The intent of this document is to provide stakeholders, such as provincial and territorial regulatory authorities, decision makers, water system owners and operators and consultants with guidance on the use of monitoring methods to assess the biological stability of water in distribution systems, with the objectives of minimizing public health risks in Canadian water systems.
Table of Contents
- Part A. Guidance on the biological stability of drinking water quality in water distribution systems
- Part B. Supporting Information
- B.1 Drinking water distribution systems
- B.2 Causes of water quality deterioration
- B.3 Monitoring methods and parameters
- B.4 Monitoring program
- B.5 Management strategies
- B.6 Water quality targets
- B.7 Microbial risk in buildings/premise plumbing
- B.8 International considerations
- Part C. References
- Appendix A. List of acronyms
- Appendix B. Select infectious disease outbreaks related to the drinking water distribution system
Part A. Guidance on the biological stability of drinking water quality in water distribution systems
A.1 Introduction
Water leaving a treatment facility enters an extensive network of pipes (also referred to as watermains), valves, hydrants, service lines and storage facilities, known as the drinking water distribution system, before it reaches the consumers' tap. Ideally, there should be minimal change in water quality in the distribution system. This occurs when the water is "biologically stable". For the purposes of this document, biological stability (also known as biostability) refers to the concept of providing consumers with drinking water at a low risk of supporting significant microbiological growth, such that their safety or aesthetic perception is not affected.
Distribution systems represent a complex and dynamic environment — sometimes referred to as a "reactor" — where numerous physical, chemical and biological interactions and reactions involving microorganisms, nutrients and particles, occur. This mixture forms biofilm and loose deposits which can lead to a deterioration in water quality and can result in a variety of problems, including direct (e.g., waterborne outbreaks) and other health risks (e.g., metal exposures), and aesthetic issues (e.g., colour, turbidity or unpleasant taste and odour). Despite this, water quality deterioration occurring during distribution is generally not characterized or well-understood.
A.2 Scope and Aim
The intent of this document is to provide responsible authorities, such as municipalities and water system operators, with an overview of: 1) causes of microbial water quality deterioration in the distribution system; 2) monitoring tools that can be used to assess biological stability; and 3) distribution system management strategies. Although the primary focus of this document is on the component of the distribution system that carries water to buildings, there is a brief discussion of premise plumbing. It is acknowledged that a water utility's responsibility does not generally include plumbing systems.
The guidance presented here replaces the Guidance on the Use of Heterotrophic Plate Counts in Canadian Drinking Water Supplies (Health Canada, 2012).
A.3 Causes of water quality deterioration
Water quality deterioration in the distribution system is due to a multitude of factors and mechanisms. Table 1 outlines select factors and mechanisms leading to deterioration in microbial water quality.
Factor | Effect on microbial water quality in the distribution system |
---|---|
Presence of microorganisms | Microorganisms are present in all drinking water distribution systems. The majority of these microorganisms are attached to the inner walls of pipes, as part of biofilms and/or loose deposits, where they are protected from disinfectants and other threats. |
Type and availability of nutrients | A number of nutrients are present in drinking water distribution systems, and can promote microbial growth, either by serving as fuel for microorganisms or by consuming disinfectant residual. Biofilm and loose deposits constitute a large reservoir of organic nutrients, at concentrations far exceeding those in bulk water. |
Temperature | Water temperature is one of the most important factors influencing microbial dynamics in the distribution system. Warmer water temperatures can lead to increased microbial growth, either directly, or via accelerated decay of disinfectant residuals. Temperature fluctuations can also affect microbial attachment. |
Pipe material and condition | Biofilms and loose deposits accumulate in all distribution systems regardless of pipe material. However, biofilm biomass tends to be lower on plastic pipes compared to iron. In addition to pipe material, pipe condition can drastically affect water quality in distribution systems. As pipes age, they become prone to leaks and breaks, and more vulnerable to intrusion of contaminants. |
Type and concentration of disinfectant residual | Disinfectant residuals possess different capabilities in terms of disinfectant power, reactivity with organic and inorganic material, biofilm penetration, and potential for disinfection by-product formation. Regardless of the type of residual disinfectant used, decreases in concentration in the drinking water distribution system are associated with increased (re)growth. |
A.4 Monitoring methods and parameters
Given the "reactor" nature of drinking water distribution systems, it is essential to monitor changes in biological stability, in order to minimize potential risks to consumers. This has traditionally been done using bacterial indicators (e.g., total coliforms and E. coli) and heterotrophic plate count (HPC) monitoring. While these methods are useful and provide information regarding water quality changes that may impact biological stability, they suffer from significant limitations. A variety of other monitoring tools can be used. In this guidance document, monitoring has been categorized as either: 1) basic, 2) operational or 3) advanced in nature. Basic monitoring is consistent with the minimum monitoring recommended for drinking water systems serving the public. Operational monitoring provides an understanding of distribution system dynamics and the factors contributing to water quality deterioration. Advanced methods are presented for those water utilities that have the resources to study water quality in more detail; they may require partnerships between water utilities and universities or advanced commercial laboratories.
Water utilities should use the most appropriate measures, depending on resources, to establish baseline conditions, monitor changes and detect potential or actual contamination events. Monitoring plans should be based on a system-specific assessment, and meet the requirements of the responsible drinking water authority. Suggested parameters/methods to consider are presented in Table 2.
It is important for water utilities to recognize that many of the listed parameters (e.g., disinfectant residual, turbidity) should already be monitored as part of a source-to-tap approach to producing safe drinking water. Other parameters are relatively easy to use and provide rapid results. Some are advanced methods that only large systems will have the resources to apply (e.g., flow cytometry). Once data are collected, they should be analyzed to assess if, and how, distribution system water quality is changing. Water quality goals can then be established. The monitoring plan should also specify actions that should be taken if water quality goals are not met (e.g., increase disinfectant residual).
Type | Suggested parameters/methods |
---|---|
Basic | Bacterial indicators (total coliforms and E. coli) |
Disinfectant residual | |
Turbidity | |
Conductivity | |
Pressure | |
Operational | Temperature |
Microbiological activity – heterotrophic plate count and/or adenosine triphosphate | |
pH | |
Oxidation-reduction potential | |
Colour (apparent and true) | |
Nutrient concentrations | |
Metals (dissolved and particulate) | |
Biofilm formation rate | |
Corrosion rate | |
Advanced | Flow cytometry |
Molecular methods | |
Pipe autopsies and characterization of accumulated material | |
Water distribution system models |
A.5 Management strategies
A well-maintained and operated distribution system is a critical component of providing safe drinking water. It is recommended that water utilities develop a management plan to understand how the complex biological and physio-chemical interactions and reactions that occur in the distribution system impact biostability and consequently the safety of drinking water. Water utilities may require a multi-faceted approach to effectively balance concomitant objectives (e.g., water quality, physical integrity). Management strategies will be unique to each system based on their design, size and complexity, as well as regulatory requirements. Water utilities are responsible for identifying and managing the full range of risks that may apply to their system(s). Guidance is provided in Part B to assist water utilities.
Part B. Supporting Information
B.1 Drinking water distribution systems
Water leaving a treatment facility enters an extensive network of pipes (also referred to as watermains), valves, hydrants, service lines and storage facilities, known as the drinking water distribution system, before it reaches the consumers' tap. Ideally, there should be minimal change in water quality during distribution until the point of consumption. This occurs when the water is "biologically stable". The concept of biological stability was first introduced in the 1980s (Rittmann and Snoeyink, 1984), and its definition has changed with the evolution of new monitoring approaches (Sibile, 1998; van der Kooij, 2000, 2003; Lautenschlager et al., 2013; Prest et al., 2016a). For the purposes of this document, biological stability refers to the concept of providing consumers with drinking water at a low risk of supporting significant microbiological growth, such that their safety or aesthetic perception is not affected.
Achieving biological stability requires that water utilities produce biologically stable water, and that the distribution system be operated and maintained such that minimal water quality deterioration occurs. It is important to recognize that treated water is not sterile, and contains particles, nutrients, and a microbial load (Figure 1) (Liu et al. 2013a,b). Once this water enters the distribution system, numerous biological and physio-chemical interactions and reactions involving microorganisms, nutrients and particles occur (Figure 1). This is why distribution systems are sometimes referred to as "reactors". These complex and dynamic interactions lead to the formation of biofilm and loose deposits, which contain microorganisms (Figure 1). The result is that water quality can deteriorate and lead to a variety of problems, including direct and other health risks, and aesthetic issues, such as colour, turbidity or unpleasant taste and odour.
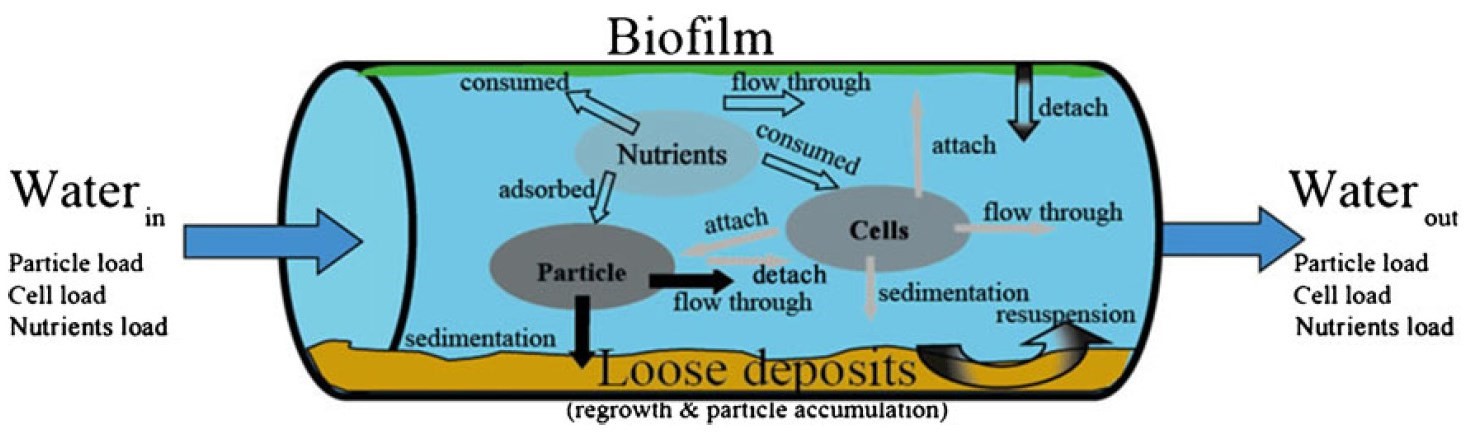
Figure 1: Text description
An illustration showing the biological and physio-chemical interactions and reactions within the drinking water distribution system. The illustration shows a pipe section of the drinking water distribution system through which water is flowing from left to right. The water flowing into the pipe carries a load of particles, cells and nutrients. Once in the pipe, these particles, cells and nutrients are shown interacting with each other and with components of the pipe itself. Particles are shown falling to the floor of the pipe to join a layer of loose deposits (aka sedimentation). Other particles are shown detaching from the pipe wall (aka resuspension), while others are shown flowing through and exiting the pipe to join the participle load leaving the right side of the pipe. Nutrients are shown being consumed by the biofilm layer attached to the top of the pipe wall, and by cells and particles. Cells are shown attaching to particles and to the pipe floor, and exiting the pipe. Biofilm is shown detaching from the pipe wall.
B.1.1 Direct health risks
The degree to which water quality deterioration in the distribution system contributes to human illness is difficult to quantify because many events are not detected or recognized. In addition, rates of endemic infectious illness — including waterborne illness — are significantly underreported and underdiagnosed, for a number of reasons (Majowicz et al., 2004; MacDougall et al., 2008; Gibbons et al., 2014). This is further complicated by the fact that, in Canada, there is no national surveillance system specific to waterborne illness and no standardized approach to data collection on sporadic or outbreak cases of waterborne illness (Pons et al., 2015). Instead, provinces and territories report notifiable disease data to the federal government (Public Health Agency of Canada, 2021) based on the disease (e.g., campylobacteriosis) rather than the route of transmission (e.g., waterborne).Thus, there is limited information regarding the magnitude and sources of waterborne illness in Canada, including those attributable to drinking water.
While Canadian surveillance data are scarce, United States (US) surveillance data clearly show a link between distribution system contamination and human illness. Between 1995 and 2014, over 40 waterborne disease outbreaks attributed to distribution system deficiencies were reported in the US (Levy et al., 1998; Barwick et al., 2000; Craun and Calderon, 2001; Lee et al., 2002; Blackburn et al., 2004; Liang et al., 2006; Yoder et al., 2008; Brunkard et al. 2011; Hilborn et al., 2013; Beer et al., 2015; Benedict et al., 2017). These resulted in over 4,800 cases of illness. A meta-analysis of US data, conducted by the World Health Organization (WHO), showed that the majority of waterborne disease outbreaks attributed to distribution system were related to cross-connections (Figure 2); and bacteria were the most common type of etiological agent (WHO, 2014; Renwick et al., 2019). The release of biofilm and deposits in the distribution system, related to a water source change, was implicated in the Legionella outbreak that occurred in Flint, Michigan, US, between 2014 and 2015 (Rhoads et al., 2017; Zahran et al., 2018).
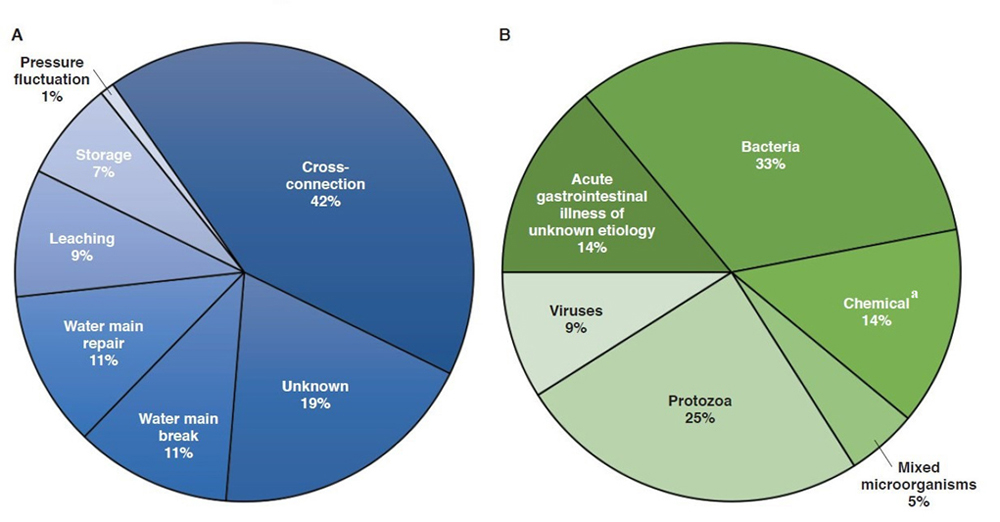
a – Outbreaks associated with chlorine, copper and lead.
Figure 2: Text description
An illustration showing two pie charts, A and B. Pie chart A illustrates the portion of distribution system-related outbreaks associated with a specific system fault. Cross-connections are the largest piece of the pie (42%), followed by: unknown causes (19%), water main breaks and repairs (each accounting for 11%), leaching (9%), storage (7%) and pressure fluctuation (1%). Pie chart B illustrates the portion of distribution system-related outbreaks associated with an etiological agent. Bacteria account for the largest piece of the pie (33%), followed by: protozoa (25%), chemical and unknown agents (each accounting for 14%), viruses (9%) and mixed microorganisms (5%).
Several international waterborne outbreaks attributable to the distribution system have also been reported (Nygård et al., 2004; Jakopanec et al., 2008; Moreira and Bondelind, 2017; Viñas et al., 2019), and are highlighted in Appendix B. They, like the US outbreaks, show that cross-connections were the main cause of outbreaks in the distribution system; and demonstrate the significant impact of distribution system water quality deterioration on health (Moreira and Bondelind, 2017). An example of this is the extensive outbreak that occurred in Nokia, Finland in 2007. Over 8,400 individuals developed gastroenteritis due to contamination of distributed water by sewage effluent that entered via a cross-connection (at the sewage treatment plant) (Laine et al., 2011; Hrudey and Hrudey, 2014; Moreira and Bondelind, 2017). In addition to cross-connections, distribution system-related outbreaks have been associated with maintenance or repair work of water mains, storage facility contamination, and intrusion of sewage due to leakage (Appendix B; Hrudey and Hrudey, 2004, 2014; Moreira and Bondelind, 2017).
Epidemiological studies have also highlighted an association between the distribution system and illness (Hunter et al. 2005; NRC, 2006; Nygård et al., 2007; Córdoba et al., 2010; Lambertini et al., 2012; Ercumen et al., 2014; Säve-Söderbergh et al., 2017; Viñas et al., 2019). Nygård et al.( 2007), for example, reported that breaks and maintenance work in the distribution system led to an increased risk of gastrointestinal illness among consumers. Similarly, Säve-Söderbergh et al.( 2017) noted a significant increase in gastrointestinal illness amongst consumers in areas where distribution system incidents, defined as temporary changes in the hydraulic pressure and physical integrity, had occurred. Pressure fluctuations (i.e., transients) represent a serious public health risk as low or negative pressure can allow contaminants to enter the distribution system (Kirmeyer et al., 2001; Besner et al., 2010, 2011).
Models used to explore public health impacts estimate that between 15 and 50% of waterborne gastrointestinal illness can be attributed to distribution system risks (Payment et al., 1991, 1997; Messner et al., 2006; Nygård et al., 2004, 2007; Murphy et al., 2016). In 2021, the US Centers for Disease Control and Prevention (US CDC) estimated that biofilm-associated pathogens, including Legionella, non-tuberculous mycobacteria and Pseudomonas, accounted for less than 1.5% of all cases of infectious waterborne illness. Despite this, these pathogens contributed a large proportion of the burden of waterborne disease (i.e., greater than 70% of hospitalizations and 90% of deaths linked to waterborne pathogens), and almost 80% of $3.3 billion USD/year of the direct healthcare costs (Collier et al., 2021).This study underscores the potential role of these microorganisms on infectious waterborne disease burden.
B.1.2 Other health risks
Metal precipitates (see Figure 1, particle accumulation), including aluminum, iron, or manganese, can act as an accumulation sink for other contaminants (e.g., arsenic, chromium, copper, lead) (Cantor, 2017). This material can be disturbed and "released" in an uncontrolled manner due to hydraulic disturbances (e.g., fire-fighting activities, watermain breaks, pump station operation) or flushing operations. Table 3 summarizes the concentrations of biological matter (also referred to as biomass) and metal precipitates measured in material removed from two full-scale surface water systems using a range of flushing velocities. Elevated concentrations of microorganisms and metals were measured. Other researchers have reported similar findings for systems using groundwater, surface water and a blend of ground/surface water (Lytle et al., 2004; Seth et al., 2004; Friedman et al., 2010a; Douterelo et al., 2016a; Li et al., 2018). Collectively, these studies demonstrate that significant biomass and metal precipitates can accumulate and lead to deterioration in water quality. This may result in human illness.
The release of microorganisms or metals is also generally associated with discolouration or turbidity events (Prince et al., 2003; Seth et al., 2004; Besner et al., 2008; Husband et al., 2016). Husband and Boxall (2010) reported that cast iron watermains consistently demonstrated higher turbidity with the release of accumulated material compared to polyethylene or polyvinyl chloride (PVC) watermains. Burlingame et al. (2006) reported a direct relationship between turbidity and the release of accumulated material from tuberculated iron pipes. Seth et al. (2004) found elevated turbidity and metals concentrations in material flushed from cast iron, PVC and polyethylene watermains. Thus, consumer complaints of colour, or unpleasant taste and odour, can serve as an indicator of water quality deterioration in the distribution system (Hrudey and Hrudey, 2014).
Community and pipe material | Velocity (ft/sec)Table 3 - Footnote a |
HPC-R2ATable 3 - Footnote b (cfu/mL) |
Total viable biomassTable 3 - Footnote c (pg/mL) |
Viable bacteriaTable 3 - Footnote d (cells/mL) |
Iron (µg/L) |
Manganese (µg/L) |
---|---|---|---|---|---|---|
Portland, Oregon – cement-lined | 4 | 930 | 9.3 | 89,200 | 4,000 | 800 |
6 | 750 | 2.7 | 28,700Table 3 - Footnote e | 4,400 | 180 | |
6 | 3,300 | 5.9 | 54,500Table 3 - Footnote e | 6,400 | 200 | |
Portland, Oregon – cement-lined with some unlined sections | 6 | 380 | 4.0 | 34,000 | 4,300 | 330 |
Portland, Oregon – unlined cast iron | 3.0 | 130 | 1.2 | 20,700 | 3,700 | 140 |
4.8 | 2,400 | 19 | 28,100 | 26,400 | 870 | |
6.0 | 430 | 2.0 | 37,900 | 15,100 | 300 | |
6.0 | 2,900 | 54 | 61,400 | 16,500 | 800 | |
6.4 | 1,030 | 4.7 | 31,300 | 7,500 | 210 | |
Seattle, Washington – unlined cast iron | 3.0 | 1,470 | 270 | 590,700 | 193,100 | 20,600 |
4.2 | 15,500 | 807 | 689,100Table 3 - Footnote e | 139,000 | 30,100 | |
5.4 | 3,300 | 430 | 577,300 | 155,700 | 18,400 | |
6.0 | 1,500 | 280 | 601,500 | 199,000 | 20,900 | |
6.0 | 10,400 | 325 | 788,300Table 3 - Footnote e | 153,300 | 11,300 | |
|
B.1.3 Distribution systems events or deficiencies
Between 2013 and 2019, watermain breaks and pressure losses in the distribution system were identified as the main reasons for issuing a boil water advisory in Canada, accounting for 72% of advisories (Figure 3). These data are based on analyses of 5,578 records of boil water advisories, issued from 7 of 14 jurisdictions (Health Canada, 2019). A large waterborne outbreak of campylobacteriosis in Norway was attributed to pressure loss and poor distribution system integrity (Jakopanec et al., 2008). The lack of watermain disinfection following repair was also a contributing factor.
Fox et al. (2016) demonstrated that contaminants external to a small leak (5 mm diameter) in a pressurized pipe could enter the pipe and be transported within the system when negative transient pressures occur. Low and negative transient pressures can occur as a result of distribution system operation/maintenance or unplanned events such as power outages or watermain breaks. Low and negative transient pressures also allow contamination to enter the distribution system from cross-connections and/or backflow from domestic, industrial or institutional facilities (Gullick et al., 2004).
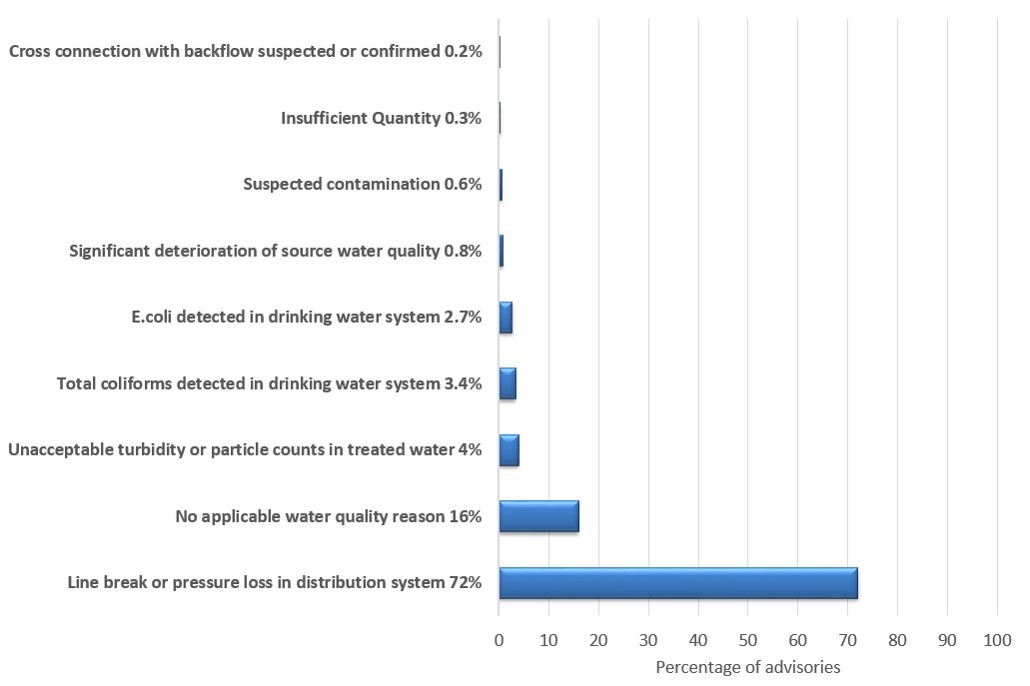
Figure 3: Text description
Figure 3 is a bar chart showing that the main reasons for issuing boil water advisories on public systems were; Line break or pressure loss in distribution system (72%), No applicable water quality reason (16%), Unacceptable turbidity or particle counts in treated water (4%), Total coliforms detected in drinking water system (3.4%), E. coli detected in drinking water system (2.7%), Significant deterioration of source water quality (0.8%), Suspected contamination (0.6%), Insufficient quantity (0.3%), Cross-connection with backflow suspected or confirmed (0.2%)
B.2 Causes of water quality deterioration
Given the "reactor" nature of drinking water distribution systems, and the resulting potential for water quality deterioration, it is important to understand the causes of this deterioration. Water quality deterioration in the distribution system is complex, and a multitude of factors and mechanisms are involved (Figures 4 and 5). A brief discussion of select factors and mechanisms leading to deterioration in microbial water quality is provided below. For a more comprehensive review, please refer to LeChevallier, 1999; van der Kooij and van der Wielen, 2014; WHO, 2014; LeChevallier et al., 2015a,b; Prest et al., 2016a,b,c.
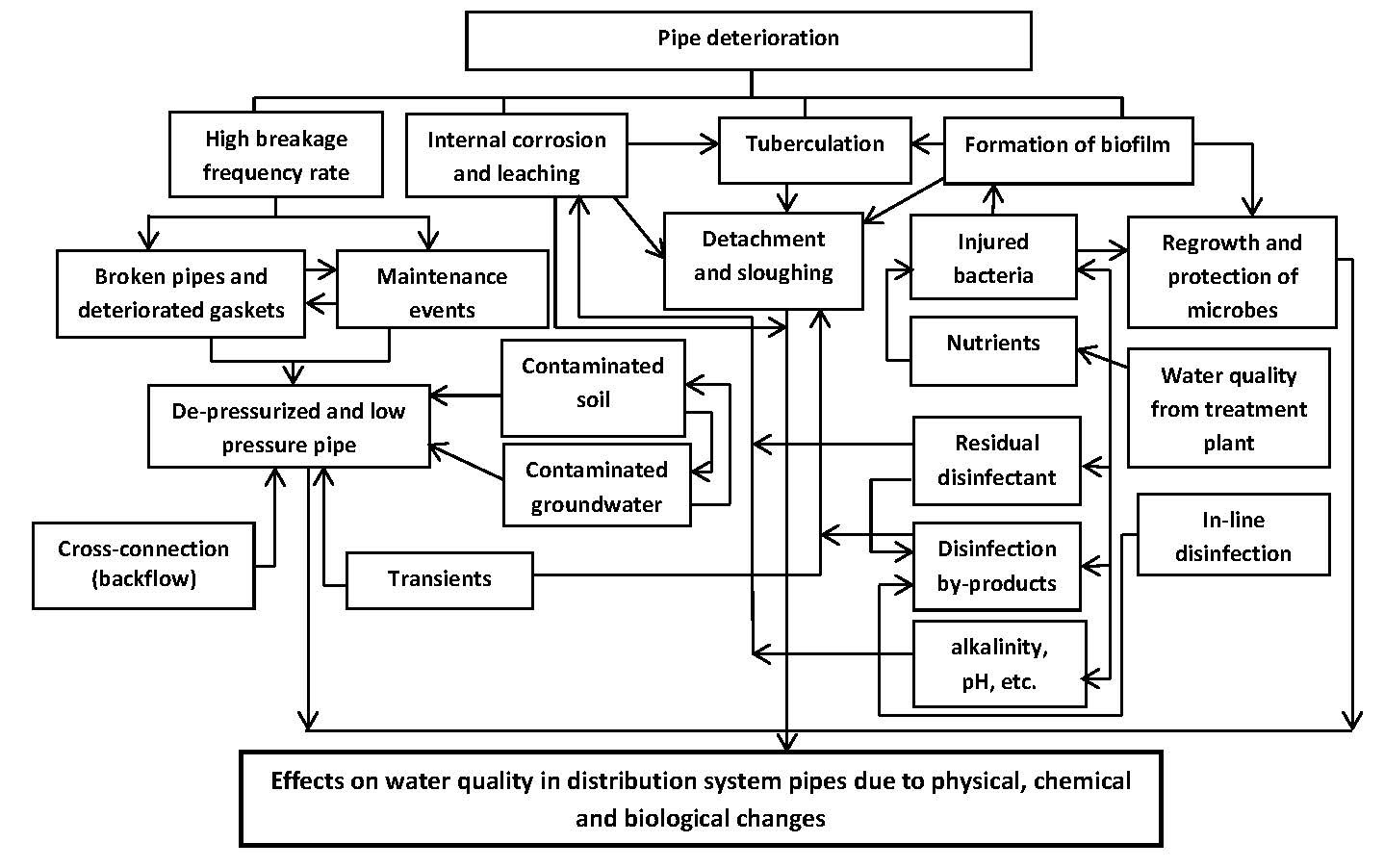
Figure 4: Text description
A flowchart illustrating the complexity of factors and interactions within the drinking water distribution system that lead to deterioration of water quality. Multiple text boxes are shown, representing a wide range of factors, and their interactions, as highlighted by arrows. A range of factor types are illustrated, including those related to: pipe condition, intrusion events, general system conditions (e.g., pH, alkalinity), and presence of microorganisms, residual disinfectant and nutrients. As an example, pipe deterioration is linked with high breakage frequency rate, internal corrosion and leaching, tuberculation and formation of biofilm. Formation of biofilm, in turn, is linked with regrowth and protection of microorganisms, detachment and sloughing, and injured bacteria. These individual factors are then shown to be linked to variety of other factors, and so on and so forth.
B.2.1 Presence of microorganisms
Microorganisms are present in all drinking water distribution systems. They are present for two main reasons: 1) they are introduced into the distribution system or 2) conditions in the distribution system favour the (re)growth of microorganisms already present. Microorganisms can enter the distribution system by surviving the water treatment process or by intrusion. Intrusion occurs when there is an integrity breach, such as a pipe break or leak, and pressure transients (LeChevallier et al., 2011). Several studies have demonstrated the potential for microbial contaminants to enter the distribution system (Karim et al., 2003; LeChevallier et al., 2003; Besner et al, 2010, 2011; Yang et al., 2011; Ebacher et al., 2012; Fontanazza et al., 2015; Fox et al., 2016). Multiple factors and mechanisms can promote microbial (re)growth, and are discussed in subsequent sections (Besner et al., 2012; Lee, 2013; LeChevallier et al., 2015a; Prest et al., 2016a,b,c; AWWA, 2017a).
The majority of microorganisms in drinking water distribution systems are attached to internal pipe surfaces (Flemming et al., 2002), as part of biofilm and/or loose deposits. The remainder exist as transient populations in the bulk water (Liu et al., 2013a,b, 2014, 2016, 2017; Proctor and Hammes, 2015) (Figures 1 and 5). Attached microorganisms can be retained for a longer period of time than transient microorganisms – i.e., years versus the time it takes for water to flow through (Liu et al., 2017). The combined genetic material of these microbial populations is known as the microbiome. Microbiomes are very heterogeneous, as well as time and site-specific both within and between distribution systems (Gomez-Alvarez et al., 2012; Chao et al., 2013, 2015; Delafont et al., 2013; Wang et al., 2014a,b; Zhang et al., 2017).
Attached microorganisms (in biofilm and loose deposits) are typically encased in a matrix of extracellular polymeric substances (EPS) that contains both organic and inorganic matter (Prest et al., 2016a; Liu et al., 2016; WRF, 2017). The EPS matrix encompasses a wide range of compounds — polysaccharides, proteins, nucleic acids and lipids — and can account for over 90% of the total organic matter in the biofilm and loose deposits (Christensen and Characklis, 1990; Flemming and Wingender, 2010; Liu et al., 2017). The EPS composition determines important properties of the biofilm such as how well it adheres to the pipe wall, how it moves under shear forces, erodes or sloughs off and how well it adsorbs dissolved and particulate substances from the bulk water (Nielsen et al., 1997; Wingender et al., 1999). The EPS structure provides protection against predators and disinfectants, and aids in uptake and utilization of nutrients (LeChevallier et al., 1988; Flemming and Wingender, 2010; Prest et al., 2016a). In addition, the polysaccharides and proteins within the EPS are important precursors to disinfection by-product formation (Wang et al., 2013).
Biofilms provide a habitat for the survival and growth of microorganisms, including pathogens (Health Canada, 2021a). A variety of enteric pathogens have been detected in biofilms (Park et al., 2001; Howe et al., 2002; LeChevallier et al., 2003; Chang and Jung, 2004; Berry et al., 2006; September et al., 2007; Gomez-Alvarez et al., 2015; Revetta et al., 2016); where they can accumulate and be released over an extended period of time (Howe et al., 2002; Warnecke, 2006; Wingender and Flemming, 2011). Non-enteric pathogens have also been detected in biofilms, including opportunistic premise plumbing pathogens (OPPPs), such as Legionella pneumophila and non-tuberculous mycobacteria (e.g., M. avium, M. intracellulare) (Norton et al., 2004; Pryor et al., 2004; Vaerewijck et al., 2005; Feazel et al., 2009; Falkinham et al., 2015; Wang et al., 2017). These organisms have adapted to grow and persist in distribution and plumbing system biofilms and have been linked to several outbreaks (Pruden et al.,2013; Beer et al., 2015; Falkinham et al., 2015; Benedict et al., 2017), including the 2014-15 Legionnaires' disease outbreak in Flint, Michigan, US (Zahran et al., 2018). They represent a significant challenge to the water industry and building managers (see Section B.7).

Figure 5: Text description
An illustration showing the microbial dynamics in a drinking water distribution system pipe. The illustration shows treated water entering a pipe section of the drinking water distribution system, and exiting at the tap. Treated water flowing into the pipe carries bacteria, particles, disinfectant residual and nutrients; and interacts with components of the pipe. Bacteria are shown consuming nutrients, attaching and detaching to/from the biofilm on the pipe wall, competing with other microbial cells, attaching to particles, and/or dying. Particles are shown falling to the floor of the pipe to join a layer of sediment, EPS and bacterial growth. Other particles are shown detaching from the pipe wall, binding to nutrients, and becoming resuspended. Nutrients are shown being consumed by the biofilm-EPS-sediment layer attached to the pipe wall and being released from the pipe wall (aka substrate leaching). Disinfectant residual is shown interacting with bacteria, leading to death and/or a microbial community shift; and interacting with the material attached to the pipe wall, along with suspended particles.
B.2.2 Type and availability of nutrients
A number of nutrients may be present in drinking water distribution systems, and can promote microbial growth, either by serving as fuel for microorganisms or by consuming disinfectant residual (NRC, 2006). The biodegradable portion of natural organic matter, referred to as biodegradable organic matter (BOM), for example, impacts distribution system water quality by providing a source of nutrients that contributes to microbial regrowth and biofilm development (Huck, 1990). Concentrations of BOM (e.g., assimilable organic carbon and biodegradable organic carbon) are only one component influencing changes in water quality in the distribution system (Prest et al., 2016a,b,c). Other nutrients have been identified as having roles in controlling microbial growth in the distribution system, including phosphorus, nitrogen, ammonia, manganese, sulphate, iron and humic substances (Camper, 2004, 2014; Coetser et al., 2005; Prest et al., 2016a,b,c). For example, Legionella requires specific nutrients for growth including iron (Percival and Williams, 2014). They can adapt to fluctuating nutrient conditions by differentiating into cell types that vary in their infectivity and resistance to disinfection (Robertson et al., 2014; NAS, 2019).
It is also important to recognize that biofilm and loose deposits (Figure 1) constitute a large reservoir of organic nutrients (Zacheus et al., 2001; Liu et al., 2013a,b) with concentrations 200 to 2 000 times higher than the bulk water (Gauthier et al., 1999). This material is available to fuel (re)growth and consume disinfectant residual (Chandy and Angles, 2001).
B.2.3 Temperature
Distribution system water quality can diminish considerably because of water temperature. In fact, water temperature is one of the most important factors influencing microbial growth (LeChevallier et al., 1990, 1996; Camper et al., 2000; van der Kooij et al., 2003; Baribeau et al., 2005; LeChevallier et al., 2015a,b; Health Canada, 2021b). In an 18-month study involving 31 full-scale systems (17 using chlorination and 14 using chloramination), higher coliform bacteria detections were reported in warmer months (LeChevallier et al., 1996). Similarly, Schleich et al. (2019) found total cell counts (measured using flow cytometry – see B.3.4.1) increased up to 5.24-fold in summer in a 12-month study of a full-scale system using chloramination.
Warmer water temperatures can also affect microbial growth via their effect on disinfectant residual. They can lead to two conflicting effects, namely: 1) increased efficacy of chemical oxidation; and 2) accelerated decay of the disinfectant residual (Li et al. 2003; van der Wielen and van der Kooij, 2010). Thus, increases in water temperature generally lead to lower biocidal effects of the disinfectant residual (Baribeau et al., 2005). LeChevallier et al. (2015a,b) observed that the biofilm formation rate was 25 times greater at temperature >15 °C compared to <15 °C in a 14-month study of six full-scale systems — three using chlorination and three using chloramination (see B.3.2.7). Elevated temperatures can also affect the solubility of metals (e.g., copper) present in the distribution systems, and result in increased leaching and corrosion (Singh and Mavinic, 1991; Boulay and Edwards, 2001; Sarver and Edwards, 2011). Temperature fluctuations can also affect biofilm attachment because of changing EPS production (Liu et al., 2016).
Climate change is expected to increase water temperature. This may exacerbate other anticipated climate-related changes, such as increased nutrient loading. This, in turn, can promote microbial growth and the survival of pathogens in distribution system biofilms. In addition, this may result in longer periods at temperatures that trigger water quality deterioration (Levin et al., 2002).
B.2.4 Pipe material and condition
Pipe material can affect microbial regrowth, and biofilm formation and composition. While biofilm can form on all pipe materials, biofilm biomass tends to be higher on corroded iron pipes as compared to uncoated plastic pipes, such as PVC and polyethylene (Niquette et al., 2000; Baribeau et al., 2005; Wang et al., 2012; Douterelo et al., 2014b; Wang et al., 2014a; Fish et al., 2016). Differences in surface characteristics including roughness and area, as well as the chemical properties of pipes, influence microbial adhesion. In the case of surface roughness for example, rougher surfaces allow microorganisms to adhere more quickly (Fish et al., 2016). Pipe material also appears to influence microbial diversity (also referred to as richness) and stability, although there is debate as to which material has the greatest impact on these parameters.
In addition to pipe material, pipe condition can significantly affect the microbial quality of drinking water in the distribution system. Pipe corrosion can generate a significant disinfectant demand, making it difficult to maintain disinfectant residual concentrations (Health Canada, 2009a). In the case of iron pipes, for example, corrosion can be exacerbated by the presence of iron-oxidizing bacteria. These bacteria are responsible for a form of microbially induced corrosion, resulting in the formation of raised outgrowths of ferrous oxide, called tubercles. Tubercles can harbour microorganisms, including opportunistic pathogens (Emde et al., 1992; US EPA, 2002; Batté et al., 2003; NRC, 2006; Teng et al., 2008); and exhibit a high disinfectant demand. The tubercles can also generate colour, turbidity, tastes and odours, as well as reduce hydraulic efficiency (Husband and Boxall, 2010).
As pipes age, they may become more prone to leaks and breaks, and more vulnerable to intrusion of contaminants (O'Connor, 2002; Moe and Rheingans, 2006; Qureshi and Shah, 2014). During low or negative pressure events, contaminants surrounding the pipes can be drawn in through leaks in the system (see Section B.1.3). Aging water infrastructure is a significant threat to water safety in Canada (Canadian Infrastructure Report Card, 2016). In Ontario, for example, many water systems were constructed in the 1960s and 1970s, (MacDonald, 2001) and, as such, will be nearing the end of their life span, which averages around 50 to 70 years (Tafuri and Field, 2010). Pipes installed during the 1960s and 1970s have also been associated with an increased likelihood of failure because of the type of material used, and poor installation practices (Besner et al., 2001; MacDonald, 2001). In other parts of Canada, pipes date back to before 1867 (Besner et al., 2001; Saint John Water, 2018).
B.2.5 Type and concentration of residual disinfectant
Residual disinfectant type and concentration also affect distribution system microbial water quality. In Canada, the majority of water utilities use free chlorine as a residual disinfectant; while the rest use chloramines (Health Canada, 2009b). These disinfectants possess different capabilities in terms of disinfectant power, reactivity with organic and inorganic material and biofilm penetration. These differences mean that the residual disinfectant is generally consumed within three days when using free chlorine compared to seven days when using chloramines (Baribeau et al., 2005). In the case of chloramines, free ammonia is released as the residual is consumed (i.e., decays); and this can lead to nitrification, the microbiological process whereby ammonia is sequentially oxidized to nitrite and nitrate by ammonia-oxidizing bacteria and nitrite-oxidizing bacteria, respectively (Wilczak, 2006). This can result in growth of nitrifying bacteria, leading to a loss in disinfectant residual and increased biofilm production, which further escalates the chlorine demand, ammonia release and microbial regrowth (Wilczak et al., 1996; Pintar and Slawson, 2003; Strickhouse et al., 2006; Wilczak, 2006; Scott et al. 2015; Bradley et al., 2020; Tolofari et al., 2020).
Regardless of the type of residual disinfectant used, decreases in concentration in the drinking water distribution system are associated with increased (re)growth (Codony et al., 2005). There is increasing recognition that higher minimum disinfectant residual concentrations are required to control (re)growth (Gagnon et al., 2008; Gillespie et al., 2014; Rand et al., 2014; LeChevallier et a., 2015a,b). Collectively, these studies indicate that disinfectant residual concentrations in the order of 1.0 mg/L free chlorine (for systems that chlorinate) and 1.8 mg/L total chlorine (for systems that chloraminate) are required to control (re)growth. LeChevallier et al. (2015b) stated that the differences between operating above and below these thresholds were stark — that is, the biofilm formation rate (see Section B.3.2.7) was six and 23 times higher when operating below the noted free and total chlorine residual concentrations, respectively.
B.3 Monitoring methods and parameters
Drinking water distribution systems are complex and dynamic environments. In order to understand changes in biological stability, a monitoring program (see Section B.4) should be designed and implemented to establish baseline conditions, monitor changes and detect on-going or potential contamination events. Comprehensive monitoring programs are recommended (Cantor, 2017, 2018; Hill et al., 2018) to obtain a better understanding of the dynamics in the drinking water distribution system, thereby increasing the likelihood of detecting periods of higher risk. Multi-parametric approaches to monitoring water quality in the distribution system are supported in the literature (Escobar and Randall, 2001; Hammes and Egli, 2005; van der Kooij, 2000; Berney et al., 2008; Vital et al., 2010, 2012; Hammes et al., 2011; Lautenschlager et al., 2013; Douterelo et al., 2014a; van der Kooij and van der Wielen, 2014; LeChevallier et al., 2015a,b; van der Kooij et al., 2015; Van Nevel et al., 2017).
For the purposes of the following discussion, potential methods or parameter analyses have been categorized as either: 1) basic, 2) operational or 3) advanced in nature.
B.3.1 Basic monitoring
The Guidelines for Canadian Drinking Water Quality: Guideline Technical Documents for Escherichia coli (E. coli) and for Total Coliforms (Health Canada, 2020a,b) recommend that bacterial indicators be monitored in conjunction with other parameters such as disinfectant residual, turbidity and pressure. Monitoring of conductivity is recommended to complement turbidity (Health Canada, 2021c). The parameters described in this section should, at a minimum, be monitored as part of a source-to-tap approach to producing safe drinking water. Once data are collected, they should be analyzed to determine their variability as discussed below.
B.3.1.1 Bacterial indicators
Routine monitoring of total coliforms and E. coli is a fundamental part of the source-to-tap approach to producing safe drinking water and forms the basis for most regulatory compliance monitoring in Canada (CCME, 2004). These indicators are used to indicate potential unsanitary conditions, physical integrity issues and (re)growth in the distribution system (Health Canada, 2020a,b). However, because they are seldom detected, they provide very little information about the microbiome (Hargesheimer, 2001; US EPA, 2016a). Hence it is recommended that they be paired with other parameters.
B.3.1.2 Disinfectant residual concentrations, turbidity and conductivity
Measuring the disinfectant residual and turbidity in the drinking water distribution system is important and should be done when bacterial indicator samples are collected (Health Canada, 2020a,b). Disinfectant residual concentration is an indirect measure of microbial abundance for both chlorinated and chloraminated systems. Decreases serve as an essential sentinel for water quality changes, such as increased microbial activity or physical integrity issues (LeChevallier et al., 1996, 1998; Haas, 1999; NRC, 2006; Nescerecka et al., 2014; Prest et al., 2016a; Health Canada, 2020a,b; Kennedy et al., 2021). Turbidity provides an indication of particulate solids in the water. A helpful corollary is conductivity, which provides an indication of the dissolved solids in the water (US EPA, 2009, 2018a; Health Canada, 2021c). These parameters should be analyzed in the field. Online or multi-parameter in-line sensors are available to conduct real-time monitoring of these and other distribution system parameters (Frey and Sullivan, 2004; LeChevallier et al., 2011; Durand et al., 2016; AWWA, 2017a; US EPA, 2018a).
Once data are collected, they should be analyzed to determine their variability. Variability, as measured by the coefficient of variation (defined as the standard deviation divided by the average for a data set) is a useful indicator of water quality stability (LeChevallier et al., 2015b). Lower values indicate less variability. Variability can also be visually assessed by graphing water quality data by sampling site as shown in Figure 6. This graph shows free chlorine residuals for eight monitoring locations in Flint, Michigan before and after the water source was changed. It clearly shows increased variability in free chlorine concentrations after the water source was changed and a return to more stable conditions with the switch back to the old source (Zahran et al., 2018). Water utilities can use their data to graph trends, establish target goals and set control limits – known as control charts (Cantor and Cantor, 2009).
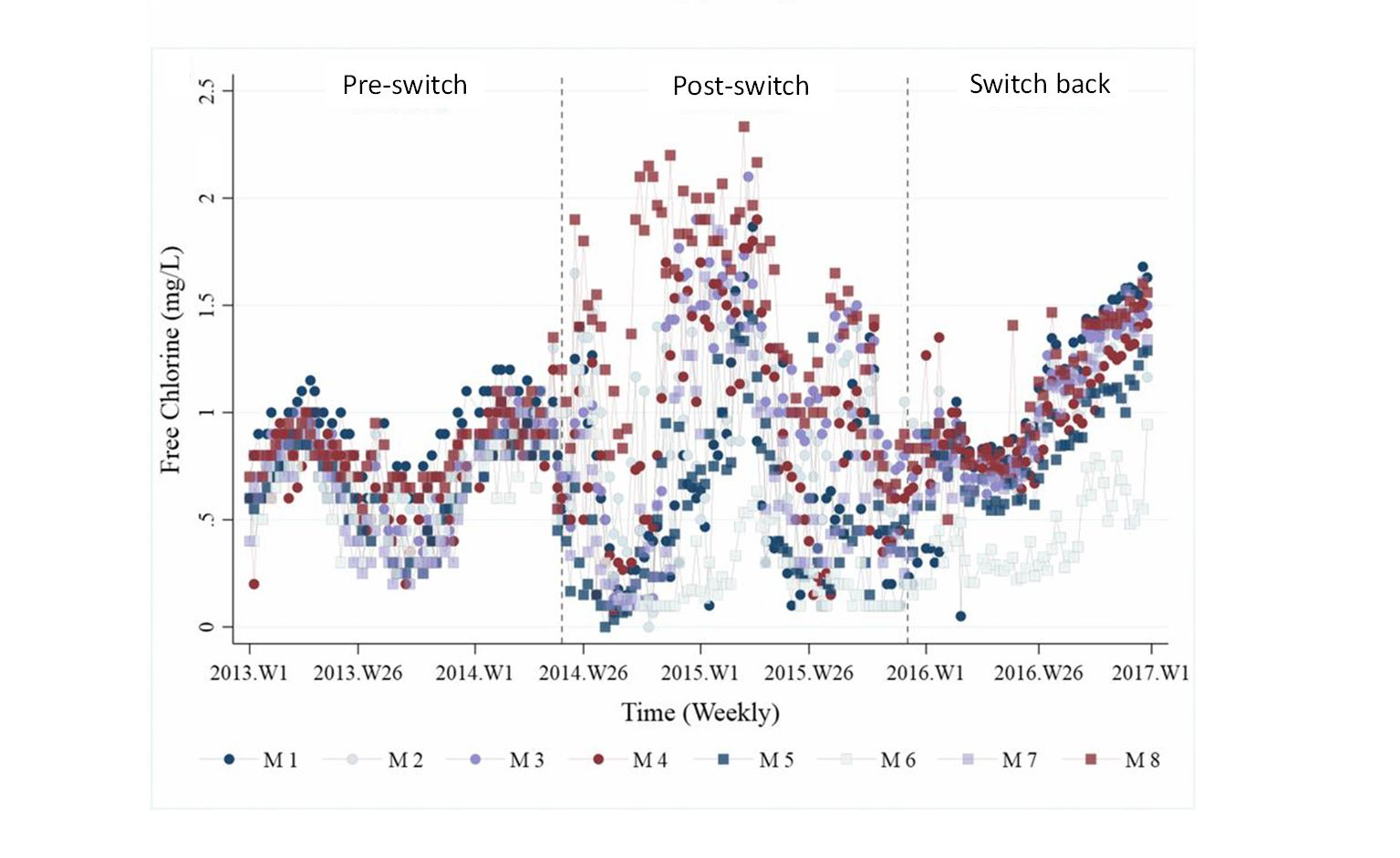
Figure 6: Text description
A scatter plot showing the free chlorine concentrations at eight monitoring stations in Flint, Michigan before and after the water source was changed. The x-axis shows the time of sampling with weekly data points between 2013 and 2016. Dashed vertical lines that indicate when the source water was changed further divide the x-axis at around the 16-week mark of 2014 and around the 40-week mark of 2015. These periods are labelled "pre-switch" "post-switch"; and "switch back". Data from eight monitoring stations is illustrated as M1 through M8. The y-axis shows the free chlorine concentration, measured in mg/L. The graph shows significant fluctuations in free chlorine concentrations after the water source was changed, and a return to more stable conditions with the switch back to the old source.
B.3.1.3 Pressure
Water pressure is a critical requirement to prevent the entry of contamination into the distribution system (Kirmeyer et al., 2001; AWWA, 2017a). As a result, guidance and/or design standards from provincial/territorial jurisdictions or industry associations outline minimum requirements (ACWWA, 2004; GLUMRB, 2012; AWWA, 2017a, 2018). Pressure fluctuations (i.e., transients) are impossible to avoid as they originate from routine activities such as a pump starting or stopping, rapid opening or closing of valves and hydrants, watermain breaks and power outages (Kirmeyer et al., 2001). Furthermore, a moderate pressure transient of 50–200 kPa (7–29 psi) can cause a watermain to fail (Rathnayaka et al., 2016).
In light of the risk associated with transients, the American Water Works Association (AWWA 2017a) recommends continuous monitoring throughout the distribution system to confirm that water pressure is within targeted ranges. AWWA (2018) recommends a minimum of two monitoring sites per pressure district — one at the site representing the lowest pressure and the other at the highest pressure. For large pressure districts, more monitoring locations may be necessary (LeChevallier et al., 2011, 2014). Advances in high-speed pressure measuring equipment (e.g., multiple readings per second) has facilitated more extensive monitoring and improved understanding of pressure transients (Friedman et al. 2004; Besner et al., 2010; Ebacher et al., 2011; Rathnayaka et al., 2016). Portable equipment also allows for increased system coverage (Sutherns, 2020; Hamilton and Nikolica, 2021).
B.3.2 Operational monitoring
It is important that water utilities integrate operational monitoring into their programs to establish baseline conditions (e.g., normal variations not requiring action), target goals and set control limits. Thus, operational monitoring is typically more comprehensive than regulatory-based monitoring (Hill et al., 2018). The parameters discussed below have been identified as useful measures for biostability (LeChevallier et al., 2015a,b; Cantor, 2017, 2018; Hill et al., 2018). Water utilities are responsible to identify the full range of operational monitoring requirements for their system(s).
B.3.2.1 Temperature
Based on the broad range of impacts that temperature can have (see Section B.2.3) and because climate change is forecast to increase water temperature, water utilities should monitor water temperature in the distribution system (Health Canada, 2021b). Thus, system-specific relationships between temperature and other parameters can be used to develop management strategies (LeChevallier et al., 2015a,b). Temperature should be measured in the field. Online instruments are also available (Buchberger et al., 2003).
B.3.2.2 Microbiological activity
Heterotrophic Plate Count (HPC)
Detection of heterotrophic bacteria has traditionally been used to assess general bacteriological water quality, including that in the distribution system (Chowdhury, 2012; Health Canada, 2012). These bacteria are naturally present in the environment, including water and are not associated with fecal contamination. They can be measured using HPCs (APHA et al., 2017). Standard HPC methods use colony formation on culture media to approximate the concentration of heterotrophic bacteria in a drinking water sample (Lillis and Bissonette, 2001; Reasoner, 2004; APHA et al., 2017). Although no single growth medium, incubation temperature or incubation time will ensure the recovery of all heterotrophic bacteria, including those that might be injured, use of R2A agar has proven most sensitive (Deininger and Lee, 2001; Uhl and Schaule, 2004; Gagnon et al., 2007; Rand et al., 2014, AWWA, 2017a).
Heterotrophic plate count can be correlated to changes in distribution system water quality (Hargesheimer, 2001; Gagnon et al., 2007; Rand et al., 2014). Unexpected increases in the HPC baseline range can indicate a disruption or contamination in the distribution system. For example, a decrease in disinfectant residual is generally associated with an increase in HPC. Despite its long history of use, low cost, and simplicity, the HPC method has several disadvantages. Among these is the requirement to not exceed an 8-hour holding time, and the time to obtain results (2-7 days). Another significant drawback is that heterotrophic bacteria represent neither the abundance nor the composition of bacteria in the drinking water (Van Nevel et al., 2017). In fact, the consensus in the literature is that the fraction of bacterial cells detected using HPC is less than one percent of the total bacterial concentration in drinking water (WHO, 2003; Prest et al., 2016a; Van Nevel et al., 2017). This means that this method greatly underestimates the concentration and diversity of bacteria present in the drinking water distribution system. Given the limitations of HPC, the water industry has been investigating alternative methods.
Adenosine triphosphate (ATP) analysis
Adenosine triphosphate measurements are gaining popularity as an indicator of total viable biomass in the distribution system (Bourbigot et al., 1982; Ochromowicz and Hoekstra, 2005; Whalen et al., 2006; Siebel et al., 2008; Hammes et al., 2010; van der Wielen and van der Kooij, 2010; Nescerecka et al., 2016; Whalen et al., 2018); assays are low cost, easy to perform, and provide results in a matter of minutes. Adenosine triphosphate is an energy molecule produced by all living organisms, and can be used as an indicator of microbial activity. A standard test method is available for detection of ATP content in microorganisms in water (ASTM International, 2015); and commercial kits, compliant with this method, are available.
The method consists of filtering water samples, followed by addition of a lysing agent in order to release cellular-ATP (cATP) from microbial cells captured on the filter (ASTM International, 2015). Luciferin-luciferase, a bioluminescence enzyme, is added, and the resulting light intensity is measured using a luminometer. The relative light units emitted are converted by comparison with an ATP standard, to provide the concentration of cATP in the sample (in pg ATP/mL) (ASTM International, 2015). This concentration is proportional to the number of viable microbial cells present in the sample. The method normally detects cATP concentrations ranging from 0.1 pg cATP/mL (i.e., detection limit) to 4 x 106 pg cATP/mL (i.e., upper limit) in 50 mL water samples (ASTM International, 2015). Kennedy et al. (2021) reported that cATP was strongly correlated to intact cell counts (measured using flow cytometry – see Section B.3.4.1) for both chlorinated and chloraminated systems. Other researchers have found similar results (Nescerecka et al., 2014; Prest et al., 2016c; Van Nevel et al., 2017).
Adenosine triphosphate measurements should be graphed and trends should be used and interpreted in conjunction with other monitoring results (Siebel et al., 2008; Hammes et al., 2010; Douterelo et al., 2014a; Nescerecka et al., 2014; Van Nevel et al., 2017). For example, ATP measurements along with disinfectant residual trends (e.g., are they decreasing), can very quickly provide an indication of increased microbial activity that requires follow-up actions. Baseline cATP concentrations will be unique to each system (Stoddart, 2020).
Cellular-ATP concentrations above 1 pg/mL have been used to trigger actions to prevent increased microbial activity in full-scale chlorinated (Hill et al., 2018) and chloraminated (Ballantyne and Meteer, 2018) distribution systems. Others have published full-scale applications of ATP measurement (Bourbigot et al., 1982; Delahaye et al., 2003; Cantor et al., 2012; LeChevallier et al., 2015a,b; Skadsen et al., 2015; Shurtz et al., 2017; McIlwain, 2020).
B.3.2.3 pH and oxidation-reduction potential
pH and oxidation-reduction potential (ORP) are critical parameters that influence the life cycle of microorganisms and the solubility of metals in the distribution system. Data from these parameters can help explain trends and variations in distribution system water quality. For example, an oxidative state (ORP >100 mV) will support aerobic microbial activity whereas a reductive state (ORP <0 mV) will encourage anaerobic microbial activity (AWWA, 2015a). An oxidative state indicates the presence of oxidizing agents such as dissolved oxygen and at high ORP values, the presence of chemical disinfectants such as chlorine (Goncharuk et al., 2010; AWWA, 2015a). High ORP indicates a water quality that is not conducive to microbial growth (Cantor, 2018).
Microbial activity can lower the pH in the distribution system due to biofilm respiration which produces carbon dioxide. This, in turn, can lead to corrosion and the release of metals (e.g., lead, copper) (AWWA, 2011). Higher pH, on the other hand, results in lower ORP. Copeland and Lytle (2014) presented the ORP for commonly used oxidizing agents under various pH conditions. For free chlorine, a concentration of ~0.2 mg/L achieved an ORP of 600 mV at pH 7 and 8 whereas at pH 9, between 0.5–0.8 mg/L was required (values interpreted from a graph). The authors also compared ORP values at pH 8 — to achieve an ORP of 600 mV, 1.1–1.7 mg/L of monochloramine was necessary compared to ~0.2 mg/L for free chlorine (values interpreted from a graph). Thus, ORP provides a rapid, single-value result that is comparable between distribution systems regardless of the disinfectant residual concentration or pH.
Overnight stagnation can trigger a change in ORP because the biofilm and loose deposits exert a chemical oxidant demand (see Section B.2.2). This, in turn, can lead to an increase in metals concentrations (Blain, 2014; Blain and Friedman, 2014; Friedman, 2014). The authors found that after 15 hours of stagnation, iron increased from <0.1 mg/L to 1.1 mg/L and manganese increased from <0.02 mg/L to 0.07 mg/L. An ORP of 700–900 mV was required to control metals concentrations; this correlated to free chlorine residuals of 0.6-0.8 mg/L. ORP versus pH relationships (known as Pourbaix diagrams) help predict the speciation of metals to better control chemically-influenced processes in the distribution system (i.e., corrosion, adsorption/desorption) (Copeland and Lytle, 2014). The WHO (2011a) recommends that the ORP necessary to ensure effective oxidation be determined on a system-specific basis.
These parameters should be analyzed in the field as changes can occur very quickly if water samples are in contact with air. Online instruments are also available (Frey and Sullivan, 2004; US EPA, 2009; US EPA, 2018a).
B.3.2.4 Colour
Colour can be associated with biofilm or metal releases (Husband and Boxall, 2010) and can be a useful indicator of water quality changes. The presence of suspended particles (e.g., clay, iron and manganese oxides) can give water the appearance of colour. Apparent colour applies to unfiltered samples and is a useful measure to assess the presence of iron and manganese oxides in the distribution system (Reiber and Dostal, 2000). A filtered sample is operationally defined as "true colour" (APHA et al., 2017) and measures colour that is due to the presence of dissolved organic matter. The comparison of apparent and true colour can help water utilities determine if colour complaints are due to suspended particles or dissolved organic matter (Health Canada, 2020c).
Online, portable and bench top analyzers are available to measure colour continuously, in the field or at the laboratory.
B.3.2.5 Nutrient concentrations
As nutrients fuel microbial (re)growth and biofilm development, water utilities should aim to minimize their concentration in treated water and have a good understanding of their concentrations in the distribution system. Water utilities that chloraminate should be particularly vigilant as free ammonia is released in distribution and premise plumbing systems as the residual decays; this can lead to significant (re)growth (Strickhouser et al., 2006; Bradley et al., 2020; Tolofari et al., 2020).
It is recommended that total or dissolved organic carbon be monitored (LeChevallier et al., 2015a,b; Cantor, 2017; Hill et al., 2018). For water utilities that chloraminate, it is important to monitor for nitrification events (e.g., total and free ammonia, nitrite, nitrate). For water utilities using phosphate-based corrosion inhibitors, monitoring throughout the distribution system is necessary to ensure a consistent corrosion inhibitor concentration. Online and portable analyzers are available to obtain rapid results.
B.3.2.6 Metals
The complex and dynamic environment found within distribution systems results in metal precipitates being bound into the biofilm and loose deposits. Changes in water quality conditions (e.g., disinfectant residual, ORP, pH) and hydraulic disturbances (e.g., hydrant flushing, watermain breaks, leak repair, firefighting activity) can cause an increase in metals concentrations.
At a minimum, monitoring should be conducted for metals that are major accumulation sinks (e.g., aluminum, iron and manganese) for other health-based contaminants. In addition, it is recommended that key health-based contaminants that are known to accumulate be monitored (e.g., arsenic, lead and any other site-specific parameters for which treatment is in place). Some laboratories offer a long list of metals for one price per sample. In this case, the full scan of metals is recommended to obtain useful information regarding scale formation and dissolution (Cantor, 2017). Online and portable analyzers are also available to obtain rapid results.
Determining the concentration of both the dissolved and particulate fractions is recommended (Cantor, 2017). Knowing whether metals are present in dissolved versus particulate form is helpful to assess the fate and transport of metals within the distribution system and to diagnose potential mechanisms leading to upsets or release events. For example, an increase in particulate metals concentrations suggests the need for watermain cleaning (e.g., unidirectional flushing) to remove hydraulically-mobile material. An increase in dissolved metals concentrations may require tighter control over treated water quality (e.g., pH, phosphate).
In order to determine dissolved metals concentrations, samples should be filtered at the time of collection (not at the laboratory). If this is not possible, the sample should be collected and delivered to the laboratory without delay for filtering and acidifying (APHA et al., 2017). For distribution system monitoring, it is acceptable to consider the particulate form to be the difference between the total and dissolved metal concentration.
B.3.2.7 Biofilm formation rate and corrosion rate
The biofilm formation rate assesses the rate and extent of (re)growth — aerobic or anaerobic — that occurs on coupons placed in a flow-through apparatus such as the one shown in Figure 7 (van der Kooij, 1999; van Lieverloo et al., 2012; LeChevallier et al., 2015b; Hooper et al., 2019). Metal coupons can be used to simultaneously measure the corrosion rate since corrosion control is necessary to minimize water quality deterioration (LeChevallier et al., 2015b; Cantor, 2017). Alternatively, coupons made of glass (van der Kooij, 1999) or polycarbonate (Hooper et al., 2019) can be used to only measure the biofilm formation rate.
The apparatus shown in Figure 7 provides a simple, easy and cost-effective way to compare the (re)growth and corrosion rate at different locations in the distribution system where it can be installed (e.g., pump station, public buildings). Water flows across the coupon at a controlled flow rate and for a set time to allow for comparisons between sites. Microorganisms attach, form a biofilm and trigger water quality changes. For the biofilm formation rate, the coupons are collected after two weeks and the quantity of ATP is measured (Hooper et al., 2019). For the corrosion rate, measurement options include monthly coupon weight loss and/or linear polarization resistance using mild steel electrodes (LeChevallier, 2015a,b). The corrosion rate should be assessed over an extended period of time, not for short term changes (AWWA, 2017b).
System-specific relationships between the biofilm formation and corrosion rates can then be established with disinfectant residual and temperature (LeChevallier et al., 2015a,b).
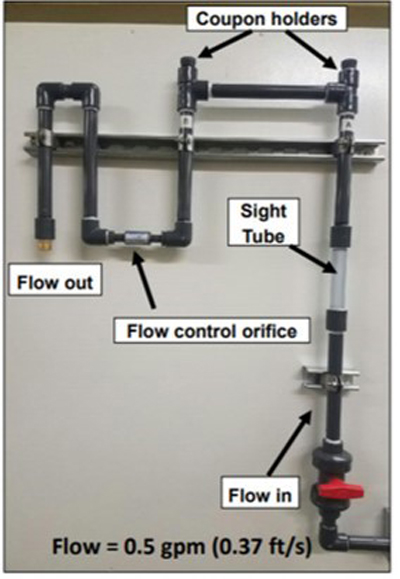
Figure 7: Text description
A photograph of a simple flow-through apparatus that can be installed at various locations in the distribution system, in order to measure microbial (re)growth and corrosion rate. The apparatus consists of a small-diameter pipe with a loop. At the bottom right of the apparatus, there is a flow control valve labelled "flow in". Moving up the pipe, a clear portion is labelled "sight tube". Further up from the sight tube is a connector pipe section labelled "coupon holders". Immediately to the left of this connector section, the pipe dips down to create a U-shape. There is a flow control orifice in the middle of the loop. At the top of the loop is a small connector that leads to pipe labelled "flow out". The flow rate is 0.5 gpm (0.37 ft/s).
B.3.3 Advanced methods
B.3.3.1 Flow cytometry (FCM)
Flow cytometry is the most established research method for monitoring of microbial water quality in the distribution system (Douterelo et al., 2014a; Van Nevel et al., 2017; Safford and Bischel, 2019), and has been applied to the study of multiple full-scale systems (Lautenschlager et al., 2013; El-Chakhtoura et al., 2015; Prest et al., 2016c; Nescerecka et al., 2018; Schleich et al., 2019; Favere et al., 2020; Kennedy et al., 2021). This method characterizes and quantifies suspended particles, including microbial cells, using an instrument called a flow cytometer. In short, particles, including microbial cells, in a sample are stained through the addition of a fluorescent dye (e.g., SYBR Green I), and this sample is then injected into the flow cytometer. Once in the flow cytometer, particles pass, one at a time, through a laser beam (Shapiro, 2003; McKinnon, 2018; Figure 8). The laser beam excites fluorescent particles, which then emit light at a higher wavelength.
Flow cytometry data can by analysed in different ways, using various "gating" strategies. Gates are placed around populations of cells with similar characteristics, in order to investigate and quantify them. Flow cytometric cell counts are reported as either total cell counts and/or intact cell counts. Intact cell counts are determined when additional staining is done, using nucleic acid-binding dyes, such as propidium iodide (PI), in order to distinguish between intact cells and membrane-damaged cells (Ramseier et al., 2011).
While there appear to be many advantages to using FCM, including that it provides the most accurate representation of the microbiome (Van Nevel et al., 2017; Kennedy et al., 2021); there are also a number of disadvantages associated with its use (Table 4). Interpretation of flow cytometry results, for example, is complicated because of the wealth of data generated and the lack of standardized analysis methods (Hammes and Egli, 2010; Van Nevel et al., 2017). Another drawback is the need to establish FCM baseline counts (i.e., those obtained during normal conditions) (Besmer et al., 2014). This necessitates widespread and long-term monitoring of the drinking water distribution system to determine flow cytometric cell counts under various conditions, and during different seasons (Besmer et al., 2014, 2016). Thus, application of flow cytometry for routine monitoring of the drinking water distribution system requires at least a few years of gathering data, in concert with other microbial monitoring methods, in order to accurately interpret results (Van Nevel et al., 2017). In addition, FCM is costly compared to other monitoring approaches, particularly due to the cost of the instrument.
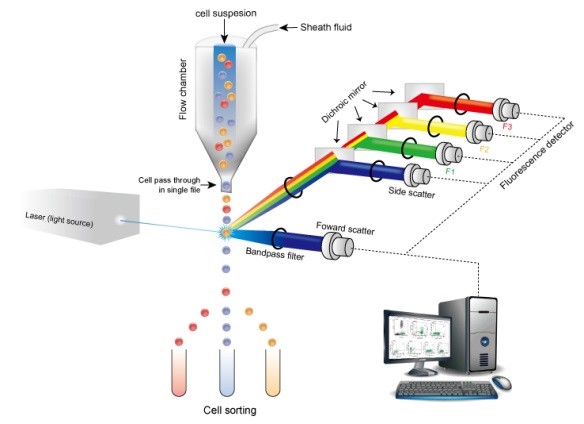
Figure 8: Text description
An illustration showing the principles of flow cytometry. The illustration shows a (stained) cell suspension, represented by a liquid made up of a mixture of small red, yellow and blue spheres, entering a funnel-like apparatus, called a flow chamber. The spheres (cells) exit the chamber in single file – i.e., one at a time. As each sphere (cell) exits, it is shown passing through a beam of light from a laser, shown as a box labeled "light source". The sphere (cell) is shown becoming excited, as represented by a burst of light, and emitting fluorescence – a rainbow of red, yellow, green and blue. This process is magnified on the right side of the illustration, where colors are shown as separate beams of light that link back to a fluorescence detector, represented by a thin cylinder (for each color) with a grey top hat, and separated by mirrors. Like-colored spheres (cells) are shown entering the same test tube after their fluorescent is recorded, as represented by an image of a computer.
Advantages | References |
---|---|
Able to measure changes in bacterial cell counts | Lautenschlager et al., 2013; Prest et al., 2013, 2016a,b,c; Nescerecka et al., 2014; Kennedy et al., 2021 |
Rapid (~15 minutes), accurate and quantitative | Van Nevel et al., 2017 |
Highly reproducible (e.g., relative standard deviations less than 2.5 for a single operator and machine) | Hammes et al., 2008; Wang et al., 2010; Prest et al., 2013; Kennedy et al., 2021 |
Able to determine viability by using nucleic acid-binding dyes | Ramseier et al., 2011 |
Amenable to automation which allows for high throughput (i.e., multi-well plate analysis feature permits analysis of up to 500 samples within a day) | Van Nevel et al., 2013 |
Online technology allows continuous FCM measurements for several subsequent weeks | Hammes et al., 2012; Brognaux et al., 2013; Besmer et al., 2014; Prest et al., 2013, 2016a,b,c |
Detailed characterization of bacterial communities using FCM fingerprints | De Roy et al., 2012; Prest et al., 2013; Koch et al., 2014; Van Nevel et al., 2017; Favere et al., 2020 |
FCM fingerprints permit increased sensitivity in detecting small changes and shifts within the bacterial community, and consistent with 16S rRNA gene analysis | De Roy et al., 2012; Prest et al., 2013; Koch et al., 2014; Props et al., 2016 |
Disadvantages | References |
Considerable requirements for equipment, user training, and data processing | Hammes and Egli, 2010 |
Subjective counting process (i.e., manual gating) | Hammes and Egli, 2010; De Roy et al., 2012; Aghaeepour et al., 2013; Prest et al., 2013 |
Does not discriminate between single cells or clumps (e.g., sloughed biofilm), potentially leading to undercounting | Shapiro, 2003; van der Kooij and van der Wielen, 2014 |
Standardized methods have not yet been developed for drinking water applications | Hammes and Egli, 2010; Lautenschlager et al., 2013; Prest et al., 2013 |
B.3.3.2 Molecular methods
A variety of molecular methods are available to assess microbial community diversity in drinking water distribution systems (Norton and LeChevallier 2000; Eichler et al. 2006; Henne et al. 2012; Pinto et al. 2012; Liu et al., 2013c, 2014, 2018; Prest et al., 2013, 2014, 2016a,b,c; Vierheilig et al., 2015; Ling et al., 2016; Van Nevel et al., 2017; Douterelo et al., 2018; Garner et al., 2021). These methods generally rely on detection, quantification and comparison of nucleic acid [Deoxyribonucleic acid (DNA) or Ribonucleic acid (RNA)]. The quantitative (q) polymerase chain reaction (PCR), also referred to as real-time PCR, is widely used for enumeration of gene targets within microorganisms present in the distribution system. This method involves processing water or biofilm samples in order to isolate DNA and/or RNA. In the case of biofilm, samples can be collected in a variety of ways, including: cut-outs of distribution pipes (LeChevallier et al., 1998; Wingender and Flemming, 2004, 2011), coupons inserted into pipes (Douterelo et al., 2016b), material mobilized into bulk water after flushing (Douterelo et al., 2014b, 2016a), household water meters (Hong et al., 2010; Ling et al., 2016), or coupons placed in a flow-through apparatus (see Section B.3.2.7).
Extracted nucleic material is then amplified using primers targeted at specific marker genes. In the case of bacteria and other prokaryotes, the 16s rRNA gene is the most widely used gene marker, whereas the18S rRNA and internal transcribed spacer genes are used for fungi and other eukaryotes (Bokulich and Mills, 2013; Bradley et al., 2016; Lan et al., 2016). Fluorescently-labelled oligonucleotide probes are also added. When these probes bind to double-stranded DNA, they fluorescence. Thus, as the target region is amplified, the emitted fluorescence is measured in real time, thereby allowing quantification of the PCR products.
Sequencing, whether it be marker gene regions or entire genomes, can provide useful information regarding the composition of microbial communities in the distribution system. A variety of next generation sequencing (NGS) technologies/platforms are available, allowing for rapid sequencing and thus, more timely and precise identification of microorganisms (Garner et al., 2021). While these and other molecular methods have several advantages, they also suffer from some significant shortcomings (Table 5), including their inability to distinguish viable from nonviable microorganisms.
Advantages | References |
---|---|
Cultivation-independent | N/A |
Allow for additional (future) analyses by freezing extracted nucleic acid | Van Nevel et al., 2017 |
NGS technologies permit real-time sequencing | Tan et al., 2015; Goordial et al., 2017; Garner et al., 2021 |
Can be used for source tracking (i.e., determining origin of contamination) | Liu et al., 2018 |
Disadvantages | References |
Inadequate detection limit (i.e., dependent on target gene and sequence length) and difficulties with viability assessment | Nocker et al., 2007, 2017 |
Time-intensive nucleic acid extraction | Nocker et al., 2007, 2017; Hwang et al., 2011, 2012; Salter et al., 2014 |
PCR amplification bias (i.e., choice of target and primers) | Nocker et al., 2007, 2017; Hwang et al., 2011, 2012; Salter et al., 2014 |
Varying assumptions and approaches to extraction, and fingerprint/sequence analysis and interpretation | N/A |
Costly and requires specialised molecular biology training | N/A |
B.3.3.3 Pipe autopsies and characterization of accumulated material
Information on the nature and quantity of material that accumulates in the distribution system can be obtained by collecting samples during pipe autopsies or when cleaning the distribution system (e.g., unidirectional flushing) (Carrière et al., 2005; Poças et al, 2013; Friedman et al., 2016). Sample analyses will depend on the source water and the study objectives. Areas to be studied can be selected based on consumer complaints, water quality concerns, disinfectant residual concentration, watermain characteristics (e.g., material, size, looped or dead-end), frequency of watermain breaks or where infrastructure is being replaced (Halton, 2001; Friedman et al., 2003; Meteer, 2018).
Pipe autopsies involve removing a section of watermain from the distribution system and characterizing the accumulated material (Halton, 2001; Muylwyk and MacDonald, 2001; Meteer, 2018). Meteer (2018) recommends performing successive scraping with a finger (protected with a silicon glove) and then with silicone, plastic and metal scrappers (in that order). Observations should be recorded and photos should be taken. Samples of the accumulated material should then be collected. Hydraulically-mobile deposits can be collected when conducting unidirectional flushing or another cleaning technique that involves a flow discharge. Hydrant nets are typically used to collect the hydraulically-mobile material at the point of flow discharge (Friedman et al., 2003).
The material collected can be characterized for a myriad of parameters as follows (Halton, 2001; Friedman et al., 2010a, 2016; Poças et al., 2013; Douterelo et al., 2014b; Hill et al., 2018; Meteer, 2018):
- physical composition (e.g., particle density and size, total suspended solids, volatile solids);
- chemical composition (e.g., metals, ions, nutrients);
- biological composition using common methods (e.g., ATP, HPC-R2A, total coliforms, iron-reducing bacteria, sulphur-reducing bacteria); and
- biological composition using advanced methods (e.g., viable bacteria using flow cytometry – see Section B.3.2.1, microbial community analysis – see Section B.3.4.2).
Carrière et al. (2005) recommends calculating the deposit accumulation rate to establish optimal cleaning frequencies and identify alternatives to limit the build-up of deposits in the distribution system (e.g., enhanced removal of material at the treatment facility, optimized coagulation/flocculation to minimize post-precipitation in the distribution system, corrosion control).
B.3.3.4 Water distribution system models
Small-scale physical models such as pipe rigs or pipe loops can be used to study how changes in water chemistry impact water quality and to evaluate mitigative measures (Health Canada, 2009b; Cantor, 2012, 2017, 2021; Friedman, 2014). These models generally provide insight into a myriad of factors related to biostability, including discolouration, scale formation and dissolution, microbiologically influenced corrosion and effects of operations.
Water distribution system computer models can aid in understanding changes in water quality, and in developing monitoring and sampling approaches (Skadsen et al., 2008). These computer models take into consideration the hydraulics of the distribution system, along with other characteristics to simulate distribution system dynamics (e.g., system pressures, pipe velocities, flow direction, water age, water blend ratios) (Kirmeyer et al., 2000; Powell et al., 2004; Friedman, 2020; Hatam et al., 2020). Computer models also allow water utilities to predict system behaviour under specified conditions or evaluate alternatives to address water quality issues (Powell et al., 2004; Speight and Khanal, 2009). A number of models are available, including the US Environmental Protection Agency (US EPA)'s open-source drinking water distribution system model, referred to as EPANET (US EPA, 2018b). An EPANET module, referred to as EPANET-MSX (Multi-Species Extension), considers interactions between substances found in the bulk water and on the pipe walls (e.g. disinfectants, microorganisms). Hydraulic modeling is now relatively common (Shurtz et al., 2017) although it is critical that water utilities maintain a calibration program when using computer models (Clark et al., 2010). Water quality modeling remains complicated and challenging to apply (Speight, 2021).
Quantitative microbial risk assessment (QMRA) models can also be used in conjunction with hydraulic models to better understand the risks associated with deteriorating microbial water quality in distribution systems (Blokker et al., 2014, 2018). However, obtaining accurate input data to make results more meaningful remains challenging (Besner et al., 2011; Viñas et al., 2019).
B.4 Monitoring program
B.4.1 Comprehensive monitoring program
It is important to recognize that distribution systems differ significantly in their design, size and complexity. No single monitoring program will meet the needs of all systems because of differences in spatial aspects (e.g., entry point, mid- and far-points, pressure zones), temporal aspects (e.g., diurnal or seasonal variations) and/or vulnerable sites (e.g., susceptible to intrusion, large or at-risk populations, areas with a high number of customer complaints) (Lindley and Buchberger, 2002; Hill et al., 2018). Therefore, water utilities should develop a monitoring program based on a system-specific assessment, and consider the cost and ease-of-use of monitoring methods, as well as the requirements of the responsible drinking water authority.
The system-specific assessment should establish monitoring objectives and confirm what needs to be monitored (AWWA, 2005). These elements will influence other considerations related to sample collection, location and frequency. For example, short duration events are unlikely to be captured using daily grab samples. Continuous water quality monitors increase the likelihood of detecting these events provided they are located in areas with temporal variation; storage facilities, in particular, can play an important role in affecting temporal water quality variability (Speight, 2010).
B.4.2 Sample collection
A variety of sample collection methods are available (see Table 6). Depending on the monitoring objectives, continuous online equipment or grab samples may be selected (EPA, 2018a).
Parameter | Online/data logger | Grab – field | Grab – laboratory | Other |
---|---|---|---|---|
Bacterial indicators | No | No | Yes | No |
Disinfectant residual | Yes | Yes | No | No |
Turbidity | Yes | Yes | No | No |
Conductivity | Yes | Yes | No | No |
Pressure | Yes | No | No | No |
Temperature | Yes | Yes | No | No |
HPC | No | No | Yes | No |
cATP | Yes | Yes | Yes | No |
pH | Yes | Yes | No | No |
ORP | Yes | Yes | No | No |
Colour | Yes | Yes | Yes | No |
Nutrients | Yes | Yes | Yes | No |
Metals | YesTable 6 - Footnote b | YesTable 6 - Footnote b | YesTable 6 - Footnote b | No |
Biofilm formation rate | No | No | No | Yes – flow-through apparatus and coupons |
Corrosion rate | No | No | No | Yes – flow-through apparatus and metal coupons; linear polarization resistance using mild steel electrodes |
|
B.4.3 Sampling frequency
The sampling frequency ultimately depends on a system-specific assessment of the distribution system, including its size, complexity and temporal variability, in combination with any requirements established by the responsible drinking water authority. For example, if the intent is to quickly detect a water quality change, then sampling frequency should be high (Buchberger et al., 2003). Also, distribution system water temperatures of 15 °C may trigger an increase in sampling frequency from weekly to daily for some parameters (Health Canada, 2021b).
Guidance on sampling for bacterial indicators (i.e., E. coli and total coliforms) in the distribution system is available in Canada (Health Canada, 2020a,b) and globally (US EPA, 2013; WHO, 2011a, 2014). Water utilities should assess which parameters can be measured in the field or sampled when collecting bacterial indicator samples. To establish baseline conditions, sampling on a weekly (Cantor, 2017; Hill et al., 2018) or daily basis if practical (Friedman and Slabaugh, 2020) is recommended.
Event-based monitoring should also be conducted following hydraulic disturbances (e.g., watermain break, repair or flushing) or changes in water chemistry (e.g., changes to pH, disinfection residual type, source water type), as well as when discolouration of water has been reported (Friedman et al., 2016). Some samples should be collected from sites within the distribution system (such as hydrants or valves), as well as from drinking water taps in public or private buildings to help determine the cause of the event and at-the-tap concentrations.
Practical guidance on monitoring and evaluating distribution system water quality is available in Cantor (2018).
B.4.4 Sampling locations
Careful consideration should be given to identifying locations within the drinking water distribution system where the risk of water quality deterioration is likely highest. This requires an understanding of the distribution system through a detailed description of the location of major transmission components and distribution mains. Distribution system layout details, such as the location of storage facilities, pumps, valves, meters and consumer connections, need to be considered. Additional system attributes, including pipe material, age and breakage records, are important to capture when determining where to sample. Historical water quality data are also integral to informing selection of monitoring locations (e.g., sites where contamination previously occurred). It is also important to consider system hydraulics (e.g., average water age compared to dead-ends and high water age). The types of buildings (e.g., schools, hospitals) supplied by the distribution system should also be considered, as they represent a higher number of potential exposures.
In addition to distribution system characteristics and water quality data, selection of monitoring locations will depend on the requirements of the responsible drinking water authority. Thus, the responsible drinking water authority should be consulted when identifying sampling locations.
In general, the entry point to the distribution system and points near dead-end zones and poor hydraulics (i.e., high water age) should be targeted for monitoring (Islam et al., 2015; Cantor, 2017). For metals, areas with variable turbidity (e.g., particulate solids in the water) or conductivity (e.g., dissolved solids in the water) should be targeted. In the absence of turbidity and conductivity data, sites where bacterial indicator samples are collected can be used in the interim. Once possible sampling locations have been identified, it is important to ensure that they are spatially representative (Vital et al., 2012; Nescerecka et al., 2014; Hill et al., 2018). Sample locations can be refined as water quality data is collected and trends are assessed.
B.4.5 Data analyses and response
Data generated should be tracked to evaluate trends and variability. This will allow systems to determine baseline water quality conditions (e.g., normal variations not requiring action), and thus, identify if, and how, the biological stability is changing in the distribution system. Water utilities can then establish water quality goals for the distribution system. System-specific alert and action limits should also be established to trigger preventive or corrective actions (AWWA, 2005; Cantor and Cantor, 2009). Standard operating procedures should clearly outline what actions are necessary to avoid water quality deterioration (i.e., preventive action) and to address a potential adverse water quality condition (i.e., corrective action). The objective of tiered response protocols is to avoid the need for emergency measures which are generally much more complex and labour-intensive (AWWA, 2005). If water quality goals are not met, the system-specific preventive or corrective actions should be taken (Ballantyne and Meteer, 2018).
More detailed information on water quality monitoring data management and analysis is available elsewhere (AwwaRF, 2002; Cantor and Cantor, 2009; AWWA, 2017a; Cantor 2018).
B.5 Management strategies
The drinking water distribution system is the last protective barrier before the consumers' tap. A well-maintained and operated distribution system is a critical component of providing safe drinking water. Although drinking water distribution systems can vary considerably, they face common challenges, including water quality deterioration (see Section B.2). In order to ensure delivery of safe drinking water to consumers, the causes of this deterioration need to be understood. It is recommended that water utilities develop a distribution system monitoring plan to identify sources of contamination and/or causes of microbial (re)growth.
Monitoring results inform the selection of appropriate management strategies. Some strategies are discussed below; water utilities are responsible to identify and manage the full range of risks that may apply to their system(s). Comprehensive reviews can be found elsewhere (NRC, 2006; Kirmeyer et al., 2014; Mosse and Murray, 2015; Cantor, 2017, 2018).
B.5.1 Water entering the distribution system
Water utilities should be aware of how source water quality and treatment processes impact the biological stability of water entering the distribution system. For example, groundwater typically has lower cell counts and less organic nutrients compared to surface water (Najm et al., 2000; Prest et al., 2016b). However, groundwater can have higher concentrations of inorganic nutrients (e.g., iron, manganese, nitrogen or sulphur) (AWWA, 2011) and sediment may accumulate in the distribution system, storage facilities, etc. (Lotimer, 2012). Also, anaerobic groundwater can have high biofilm formation rates (i.e., up 80 pg ATP/cm2-d) (van Lieverloo et al., 2012) likely due to high concentrations of methane, iron, ammonium or manganese (de Vet, 2011). Surface and subsurface sources should be characterized with regard to nutrient concentrations (Cantor, 2017).
Treatment processes can also significantly impact the quality of water entering the distribution system (AWWA, 2011). For example, oxidation processes produce biodegradable nutrients upon reaction with organic matter (Alarcon-Herrera et al., 1993; Bursill, 2001; Reckhow et al., 2007). Thus, biologically active filtration may be necessary to stabilize treated water (GLUMRB, 2012). In some European countries, the approach taken to achieve biological stability is to produce drinking water with an assimilable organic carbon of <10 μg/L to control or limit microbial activity in the absence of a disinfectant residual (van der Kooij, 2000; Smeets et al., 2009; Lautenschlager et al., 2013). In North America, minimum disinfectant residuals are typically recommended to effectively control (re)growth in the distribution system (LeChevallier et al., 1996; LeChevallier and Au, 2004) (see Section B.5.2).
In addition, the pH selected for treated water should consider chemically-influenced processes in the distribution system (i.e., corrosion, adsorption/desorption). To maintain biological stability, pH variability should be maintained within ±0.2 units throughout the distribution system (Muylwyk and MacDonald, 2001; Friedman et al., 2010a; Health Canada, 2015).
B.5.2 Disinfectant residual
It is important to ensure the disinfectant residual leaving the treatment facility is stable otherwise it may be difficult to maintain a residual throughout the distribution system (Alexander et al, 2019; US EPA, 2019). A "hold study" is a simple and cost-effective way to assess how long a disinfectant residual can be effectively maintained in the distribution system. The study involves collecting samples, "holding" them for a duration representative of the system retention time and measuring disinfectant residual decay. It can be conducted for both cold (<10 °C) and warm (>10 °C) water conditions to assess the impacts of temperature. Study findings identify where water utilities should focus their efforts (e.g., the treatment facility or the distribution system). An example of a treatment change is to improve organic carbon removal, which has multiple benefits-it reduces the disinfectant demand thereby increasing residual stability and reduces the potential for biofilm formation in the distribution system which in turn increases residual persistence (Chandy and Angles, 2001). Practical guidance is available to help water utilities carry out hold studies (Alexander et al. 2019; US EPA, 2019). Other approaches to maintain an effective disinfectant residual in the distribution system include managing water age (see Sections B.5.3 and B.5.4) and reducing the disinfectant demand associated with loose deposits (see Section B.5.5).
B.5.3 Storage facilities
Storage facilities are important infrastructure assets to maintain distribution system pressure and supply peak water demands. However, they can cause water quality deterioration if they are not properly operated and maintained (see Section B.1). Stagnation and excessive retention time can result in thermal stratification, loss of disinfectant residual, and/or (re)growth (US EPA, 2002b; Delahaye et al., 2003). For those systems that chloraminate, nitrification is often associated with storage facilities (US EPA, 2002b; Baribeau et al., 2005). Storage facility sediments have also been shown to harbour OPPPs such as Legionella spp. and Mycobacterium spp. (Lu et al., 2015; Qin et al., 2017).
In light of the risks associated with storage facilities, guidance and/or design standards to avoid stagnation and minimize retention times are available from provincial/territorial jurisdictions or industry associations (e.g., AWWA). It is also important to establish a unique monitoring program for each storage facility (e.g., operational data, sediment and biofilm sampling) to determine:
- a turnover rate to maintain target disinfectant residual concentrations and manage water age for temperatures <15 °C and >15 °C; and
- an inspection, cleaning and maintenance schedule to detect potential entry points for contamination and remove accumulated material.
Practical guidance to manage the risks associated with storage facilities is available (Kirmeyer et al., 1999; Martel et al., 2002; US EPA, 2002b). Information regarding the disinfection of storage facilities and field dechlorination is available in ANSI/AWWA (2019) and (2018), respectively.
B.5.4 Water age and hydraulic integrity
To manage water age, water utilities should aim to minimize the retention time in watermains and storage facilities (see Section B.5.3). To reduce water age in watermains, careful system design is necessary. Oversized or dead-end watermains can lead to excessive water age hence procedures should be in place to manage water quality deterioration (Kirmeyer et al., 2000; AWWA, 2011). For example, post hydrants can be installed to flush dead-end watermains (Locco and Alberton, 2021). Special precautions may apply for long transmission mains that connect two or more communities (e.g., regional systems, rural pipelines). The responsible drinking water authority should be contacted to confirm applicable requirements.
Water utilities should also have strategies that aim to minimize: 1) pressure fluctuations (i.e., transients); 2) rapid and/or extreme fluctuations in flow velocities; and 3) the frequency of flow reversals. These types of activities can cause watermain breaks (Rathnayaka et al., 2016) or stir up and entrain the material that has accumulated in the distribution system. The latter can result in discoloured water events (Prince et al., 2003; AWWA, 2011), as well as at-the-tap concentrations that exceed maximum allowable concentrations (Friedman et al., 2016). Intelligent control systems Footnote 1 can mitigate the effects of pressure transients (e.g., slow pump start/stop); this, in turn, minimizes physical and hydraulic disturbances (Blake, 2019; Steger and Pierce, 2019). Advances in high-speed pressure measuring equipment (e.g., multiple readings per second) has also facilitated the implementation of programs to reduce water leakage and watermain breaks (Chapman and Ziemann, 2019; Sutherns, 2019; Ginn and Smither, 2020; Hamilton and Nikolica, 2021). Coordination with the agencies that conduct fire flow testing can also prevent hydraulic fluctuations (AWWA, 2011).
B.5.5 Watermain cleaning
Proactive watermain cleaning can minimize the risks associated with the material that has accumulated in the distribution system. A variety of pipe cleaning strategies, aimed at removing biofilm, loose deposits and sediment, are available, including unidirectional flushing (Ellison, 2003; Bellas and Tassou, 2005, Quarini et al., 2010, Vreeburg et al., 2010; Dang et al., 2014; Friedman et al., 2016; Liu et al., 2017). Cantor (2017) stressed the importance of removing accumulated material as this measure was better correlated with lower lead and copper releases than traditional corrosion control in 12 municipal and non-municipal water systems using ground and surface water. A survey of 41 full-scale systems found the most common cleaning interval was annually regardless of the type of residual disinfectant (Baribeau et al., 2005). An optimal cleaning frequency can be determined from the deposit accumulation rate (see Section B.6) (Carrière et al., 2005).
Caution is needed when using flushing. It is important that water utilities identify and implement the most appropriate flushing technique to avoid disturbing and releasing legacy deposits into the bulk water. Improper flushing techniques can stir up and potentially spread contaminants around the flushed area or deeper into the distribution system, thus increasing public health risk. The following conditions can disturb legacy deposits: excessive flushing rate or velocity; insufficient flushing rate or velocity; lack of directional control; and, inadequate flush duration (Hill et al., 2018). Automatic flushing stations are recommended if the goal is to turnover bulk water in an area due to water age or poor circulation (Hill et al., 2018).
B.5.6 Infrastructure integrity
Infrastructure integrity relates to the ability of the distribution system to act as a physical barrier to contamination (NRC, 2006). Contamination that enters the distribution system from external sources such as cross-connections, watermain breaks or leaks can compromise water quality and lead to illness (see Section B.1). Thus, it is incumbent on water utilities to have appropriate programs and/or procedures to manage these risks as briefly described below.
The need for backflow prevention and cross-connection is well established and devices are available to mitigate this risk (NRC, 2006; AWWA, 2015b). Data indicates that water utilities with low pressures experience a greater number of backflow incidents (Lee et al., 2003). Schneider et al. (2016) determined the average monthly backflow occurrence rate to be 1.6% using reverse flow sensing meters. Areas vulnerable to backflow can be identified using water distribution system hydraulic modeling (Lindley and Buchberger, 2002; Lee et al., 2003; Schneider et al., 2016). Guidance is available to help water utilities develop a backflow prevention and cross-connection program (AWWA, 2015b).
With regards to watermain breaks, there is increasing awareness that improved response procedures are needed to protect public health (Besner et al., 2008; Kirmeyer et al., 2014; Hatam et al., 2020). Kirmeyer et al. (2014) assessed the risk of microbial contamination and tailored response procedures (e.g., boil water advisory, microbiological sampling) based on whether positive pressure could be maintained. Hatam et al. (2020) found that large volume sampling for bacterial indicators (as part of response procedures) could be appropriate at times. In addition, strict hygiene should be practiced during all watermain construction, repair or maintenance to ensure drinking water is transported to the consumer with minimum loss of quality (Kirmeyer et al., 2001, 2014). Information regarding the disinfection of watermains and field dechlorination is available in ANSI/AWWA (2014) and (2018), respectively.
A proactive leak detection program can achieve multiple benefits — less frequent leaks and watermain breaks, reduced leakage and risk of contamination, as well as asset life extension (Kachani et al., 2020). Guidance is available to help water utilities develop a program to control water loss (AWWA, 2016). Advances in high-speed pressure measuring equipment and machine learning have also improved the ability to predict watermain failures (Fitchett et a., 2020; Sutherns, 2020). "Smart" water meters can also provide flow direction, pressure and temperature data.
B.5.7 Consumer complaints
Water utilities should have programs to capture, track, analyze and resolve consumer complaints (Friedman et al., 2010b). Consumer complaints of colour, or unpleasant taste and odour, can serve as an important indicator of water quality deterioration in the distribution system (Hrudey and Hrudey, 2014). Consumers should be advised that discoloured water should not be considered safe to consume until it has been tested for metals and confirmed to be safe (Friedman et al., 2016). During watermain breaks or flushing activities, water utilities should have procedures in place to notify residents of the potential risks associated with discoloured water (i.e., elevated metals concentrations). Consumers should be advised to minimize water use to avoid sediment entering the service line. After the work is completed, homeowners should be advised to run the cold water tap in the lowest level of their residence for 5-10 minutes to eliminate any discoloured water that may have entered (Locco et al., 2018). Building owners/managers may require other precautions (see Section B.7).
B.6 Water quality targets
Water utilities should aim to maintain biologically stable water quality conditions by considering the following:
- microbially-influenced processes (Cantor, 2017);
- chemically-influenced processes such as corrosion or adsorption/desorption from materials/deposits in the distribution system (AWWA, 2011);
- physical/hydraulic changes (Besner et al., 2010; Friedman et al., 2010a,b, 2016; Cantor, 2017, 2018; Hill et al., 2018).
To achieve this, it is important that water utilities have a comprehensive monitoring program. Metrics for select parameters are summarized in Table 7. Table 7 is provided as guidance only based on the literature review that was completed to develop this document. As a result, some parameters discussed in Section B.3 may not appear in the table.
Water utilities are responsible to ensure that the distribution system is characterized and that an appropriate management plan is developed to achieve water quality goals. Water utilities may choose to use these metrics as initial water quality targets until they have sufficient data to establish system-specific values.
Parameter | Units | Systems using chlorination | Systems using chloramination | References |
---|---|---|---|---|
Disinfectant residual | mg/L | ≥ 1.0 and minimize variability | ≥ 1.8 and minimize variability | Gagnon et al., 2008 Gillespie et al., 2014 Rand et al., 2014 LeChevallier et a., 2015a,b |
TemperatureTable 7 - Footnote a | °C | 15 – biofilm formation rate 20 – corrosion rate 20 – disinfectant variability |
15 – biofilm formation rate 20 – corrosion rate 22 – disinfectant variability |
LeChevallier et a., 2015a,b |
cATP | pg/mL | <1 good control 1–10 preventive action >10 corrective action |
<1 good control 1–10 preventive action >10 corrective action |
McIlwain, 2020 |
pH | No units | Minimum 7.0 and maintain variability within ±0.2 | Minimum 7.0 and maintain variability within ±0.2 | Muylwyk and MacDonald, 2001 Friedman et al., 2010a Health Canada, 2015 |
Oxidation reduction potential | mV | >400 | >400 | Friedman, 2020 |
Dissolved organic carbonTable 7 - Footnote b | mg/L | <1.8 | <1.8 | LeChevallier et a., 2015a,b |
Biofilm formation rateTable 7 - Footnote c | pg/mm2-d | 0.090 | 0.017 | LeChevallier et a., 2015a,b |
Deposit accumulation rate | g/m/yr | <1 – flush as needed 1-10 – flush annually >10 – corrective action |
<1 – flush as needed 1-10 – flush annually >10 – corrective action |
Carrière et al., 2005 |
|
B.7 Microbial risk in buildings/premise plumbing
Premise plumbing refers to the portion of drinking water distribution system beyond the property line and in schools, hospitals, public and private housing, offices and other buildings (NRC, 2006; US EPA, 2016b). Water use in buildings includes drinking, food preparation, washing and showering, cooling systems and features (e.g., ornamental fountains).
Water quality can diminish significantly in building premise plumbing and is influenced by the same factors as those in drinking water distribution systems (see Section B.2). However, building (premise) plumbing systems face some additional challenges, including: 1) longer residence times (i.e., increased water stagnation); 2) increased water temperatures; 3) use of a variety of plumbing components and materials; 4) small pipe diameters; and 5) use of water treatment devices, such as reverse osmosis systems, that can increase corrosion. Long residence times in premise plumbing have been linked to significantly higher concentrations of microbial populations, and shifts in microbial community composition (Pepper et al., 2004; Lautenschlager et al., 2010; Manuel et al., 2010; Lipphaus et al., 2014; Bédard et al, 2018). Higher water temperatures, due to pipes being installed in heated rooms or near heat sources, promote microbial (re)growth (Lautenschlager et al., 2010; Lipphaus et al., 2014). (Re)growth is also influenced through interaction with various plumbing materials, such as plastic tubing and rubber fittings, which have considerable microbial growth promotion potential (Bucheli-Witschel et al., 2012). Smaller pipe diameters result in increased contact between microorganisms and pipes, leading to enhanced pipe material impacts (see Section B.2.3), including biofilm formation and lowered disinfectant residual concentrations (Servais et al., 1992; Rossman et al., 1994; Prévost et al., 1998). As noted earlier (see Section B.2.1), these biofilms can harbour pathogens, including OPPPs. Premise plumbing systems can dramatically enhance the growth of Legionella spp. and other OPPPs, such as Pseudomonas aeruginosa and non-tuberculous Mycobacteria, and are a significant public health concern, particularly, in hospitals (WHO, 2011b)
Premise plumbing can also impact water quality in the distribution system. The main mechanism by which microbial contamination can enter the drinking water distribution system from building premise plumbing is through backflow, either by back-siphonage or back pressure (WHO, 2011b, 2014). Thus, it is important that appropriate backflow and cross-connection control programs are in place (AWWA, 2017a).
Given the unique water quality challenges present in buildings, additional management strategies are required. It is important to note that water utilities are not generally responsible for water quality from the property line to individual points of use in buildings. Building owners or managers must monitor and manage their water systems in order to ensure safe water at the consumers' tap. Management of water quality in buildings begins with accurate and up-to-date maps of building water systems and labeling of pipework, particularly in large buildings. These are important tools to help avoid cross-connections, and identify zones were water can stagnate.
Although it is beyond the scope of this document to specify where, when and how to routinely monitor premise plumbing, some guiding principles include:
- Environmental sampling for bacteria should not occur in isolation, but as a part of a comprehensive building water management program.
- Sampling plans are unique to each building and should be based on building characteristics (e.g., size, age, layout, population served) and historical water quality data (e.g., trend analysis of previous bacterial test results, water quality parameters such as disinfectant residual and temperature).
- Water quality can vary between floors, outlets and hot and cold water taps; sites that may produce water aerosols should be considered for sampling.
- A sampling approach can be adapted based on trends and system changes.
- If indicators of potential microbial growth or other issues are detected (e.g., discoloured water, unpleasant tastes or odours and slimes in water-using devices), corrective actions such as flushing or disinfection may be needed in the building water system.
Detailed information on managing water safety in buildings is available elsewhere (WHO, 2011b; Health Canada, 2013; Public Works and Government Services Canada, 2013; ASHRAE, 2000, 2015, 2018).
B.8 International considerations
Other national and international organizations have drinking water guidance, guidelines, or standards related to monitoring water quality and biological stability in the distribution system. Variations in these can be attributed to when the assessments were completed or to differing policies and approaches. The WHO advocates a water safety plan approach that includes an operational monitoring program in the distribution system and in buildings. The WHO also suggests optimized natural organic matter removal as a means to minimize biofilm growth in the distribution system. In Australia, operational and drinking water quality monitoring parameters are defined for assessing the potential for stagnation, biofilm formation, and ingress of contamination in the distribution system. The United States US EPA's Revised Total Coliform Rule establishes routine sampling at sites throughout the distribution system, with requirements to "find and fix" sanitary defects in the distribution system. The US EPA also provides guidance on distribution system water quality monitoring in the form of various white papers and reports. The European Union's Drinking Water Directive establishes a minimum frequency of sampling in the distribution system based on the volume of water distributed or produced each day within a supply zone; and defines a series of "check monitoring" parameters.
Part C. References
ACWWA (2004). Atlantic Canada guidelines for the supply, treatment, storage, distribution and operation of drinking water supply systems. Coordinated by the Atlantic Canada Water Works Association (ACWWA) in association with the four Atlantic Canada Provinces. Halifax, Nova Scotia.
Aghaeepour, N., Finak, G., Hoos, H., Mosmann, T.R., Brinkman, R., Gottardo, R., Scheuermann, R.H., Dougall, D., Khodabakhshi, A.H., Mah, P., Obermoser, G., Spidlen, J., Taylor, I., Wuensch, S.A., Bramson, J., Eaves, C., Weng, A.P., Fortuno III, E.S., Ho, K., Kollmann, T.R. andVilar, J.M.G. (2013). Critical assessment of automated flow cytometry data analysis techniques. Nat. Methods, 10(3): 228-238.
Alarcon-Herrera, M.T., Bewtra, J.K. and Biswas, N. (1994). Seasonal variations in humic substances and their reduction through water treatment processes. Can. J. Civ. Eng., 21(2): 173-179.
Alexander, M.T., Dugan, A.G. and Wahman, D.G. (2019). Use a Hold Study to Assess Distribution System Influent Water Quality. Opflow, 45(5): 16-19.
ANSI/AWWA (2014). American National Standards Institute/American Water Works Association Standard C651: Disinfecting water mains. American Water Works Association, Denver, Colorado.
ANSI/AWWA (2018). American National Standards Institute/American Water Works Association Standard C655: Field dechlorination. American Water Works Association, Denver, Colorado.
ANSI/AWWA (2019). American National Standards Institute/American Water Works Association Standard C652: Disinfection of water-storage facilities. American Water Works Association, Denver, Colorado.
APHA, AWWA, WEF (2017). Standard methods for the examination of water and wastewater. 23rd edition. American Public Health Association, American Water Works Association and Water Environment Federation, Washington, DC.
ASHRAE (2000). Guideline 12-2000: Minimizing risk of legionellosis associated with building water systems.
ASHRAE (2015). Standard 188-2015: Legionellosis risk management for building water systems (ANSI approved).
ASHRAE (2018). Standard 188-2018 : Legionellosis risk management for building water systems (ANSI approved).
ASTM International. (2015). ASTM D4012 - 15: standard test method for adenosine triphosphate (ATP) content of microorganisms in water. In Annual book of ASTM standards. West Conshohocken, PA.
AWWA (2005). Contamination warning systems for water: An approach for providing actionable information to decision-makers. American Water Works Association, Denver, Colorado.
AWWA (2011). Water quality and treatment: a handbook of community water supplies. 6th edition. J.K. Edzwald (ed.). McGraw-Hill, New York, New York.
AWWA (2015a). Iron and manganese removal handbook, 2nd ed. American Water Works Association, Denver, Colorado.
AWWA (2015b). Backflow prevention and cross-connection control. AWWA manual of water supply practices M14, 4th ed. American Water Works Association, Denver, Colorado.
AWWA (2016). Water audits and loss control programs. AWWA manual of water supply practices M36. 4th ed. American Water Works Association, Denver, Colorado.
AWWA (2017a). Water quality in distribution systems. AWWA manual of water supply practices M68. American Water Works Association, Denver, Colorado.
AWWA (2017b). Internal corrosion control in water distribution systems. AWWA manual of water supply practices M58. Second edition. American Water Works Association, Denver, Colorado.
AWWA (2018). Self-assessment for distribution system optimization. American Water Works Association, Denver, Colorado.
AwwaRF (2002). Online monitoring for drinking water utilities. Awwa Research Foundation and American Water Works Association, Denver, Colorado.
Ballantyne, L. and Meteer, L. (2018). Nitrifying nightmares and how to avoid them. Ontario Water Works Association Annual Water Conference, Niagara Falls, ON.
Baribeau, H., Pozos, N.L., Boulos, L., Crozes, G.F., Gagnon, G.A., Rutledge, S., Skinner, D., Hu, Z., Hofmann, R., Andrews, R.C., Wojcicka, L., Alam, Z., Chauret, C., Andrews, S.A., Dumancic, R. and Warn., E. (2005). Impact of Distribution System Water Quality on Disinfection Efficacy. Report #2771. Water Research Foundation, Denver, Colorado.
Barwick, R.S., Levy, D.A., Craun, G.F., Beach, M.J. and Calderon, R. (2000). Surveillance for Waterborne-Disease Outbreaks - United States 1997-1998. Morb. Mortal. Wkly. Rep., 49:4:1.7:5:1.
Batté, M., Appenzeller, B.M.R., Grandjean, D., Fass, S., Gauthier, V., Jorand, F., Mathieu, L., Boualam, M., Saby, S. and Block, J.C. (2003). Biofilms in drinking water distribution systems. Rev. Environ. Sci. Biotechnol., 2(2-4): 147-168.
Bédard, E., Laferrière, C., Déziel, E. and Prévost, M. (2018). Impact of stagnation and sampling volume on water microbial quality monitoring in large buildings. Plos One, 13(6).
Beer, K.D., Gargano, J.W., Roberts, V.A., Hill, V.R., Garrison, L.E., Kutty, P.K., Hilborn, E.D., Wade, T.J., Fullerton, K.E. and Yoder, J.S. (2015). Surveillance for waterborne disease outbreaks associated with drinking water - United States, 2011-2012. MMWR Morb. Mortal. Wkly. Rep., 64(31): 842-848.
Bellas, I. and Tassou, S.A. (2005). Present and future applications of ice slurries. Int. J. Refrigeration 28, 115-121.
Benedict, K.M., Reses, H., Vigar, M., Roth, D.M., Roberts, V.A., Mattioli, M., Cooley, L.A., Hilborn, E.D., Wade, T.J., Fullerton, K.E., Yoder, J.S. and Hill, V.R. (2017). Surveillance for waterborne disease outbreaks associated with drinking water - United States, 2013-2014. Morb. Mortal. Wkly. Rep., 66(44): 1216-1221.
Berney, M., Vital, M., Hulshoff, I., Weilenmann, H.U., Egli, T. and Hammes, F. (2008). Rapid, cultivation-independent assessment of microbial viability in drinking water. Water Res., 42(14): 4010-4018.
Berry, D., Xi, C. and Raskin, L. (2006). Microbial ecology of drinking water distribution systems. Curr. Opin. Biotechnol., 17(3): 297-302.
Besmer, M.D., Weissbrodt, D.G., Kratochvil, B.E., Sigrist, J.A., Weyland, M.S. and Hammes, F. (2014). The feasibility of automated online flow cytometry for in-situ monitoring of microbial dynamics in aquatic ecosystems. Frontiers in Microbiology, 5.
Besmer, M.D., Epting, J., Page, R.M., Sigrist, J.A., Huggenberger, P. and Hammes, F. (2016). Online flow cytometry reveals microbial dynamics influenced by concurrent natural and operational events in groundwater used for drinking water treatment. Sci. Rep., 6: 38462.
Besner, M-C., Gauthier, V., Barbeau, B., Millette, R., Chapleau, R. and Prévost, M. (2001). Understanding distribution system water quality. J. Am. Water Works Assoc., 93(7): 101-114.
Besner, M-C., Lavoie, J., Morissette, C., Payment, P. and Prévost, M. (2008). Effect of water main repairs on water quality. J. Am. Water Works Assoc., 100(7): 95-109.
Besner, M-C., Broséus, R., Lavoie, J., Di Giovanni, G., Payment, P. and Prévost, M. (2010). Pressure monitoring and characterization of external sources of contamination at the site of the Payment drinking water epidemiological studies. Environ. Sci. Technol., 44(1): 269-277.
Besner, M-C., Prevost, M. and Regli, S. (2011). Assessing the public health risk of microbial intrusion events in distribution systems: Conceptual model, available data, and challenges. Water Res., 45(3): 961-979.
Besner, M-C., Modak, P.R. and Glauser, N. (2012). Extensive sediment characterization during unidirectional flushing in a distribution system. 14th Water Distribution System Analysis Conference, v. 2, pp. 871-876.
Blackburn, B.G., Craun, G.F., Yoder, J.S., Hill, V., Calderon, R.L., Chen, N., Lee, S.H., Levy, D.A. and Beach, M.J. (2004). Surveillance for waterborne-disease outbreaks associated with drinking water - United States, 2001-2002. MMWR Surveill. Summ., 53(8): 23-45.
Blain, A. (2014). Water quality update. Prepared for City of Longview, Washington.
Blain, A. and Friedman, M. (2014). Water quality update. Prepared for the City of Longview, Washington.
Blake, M. (2019). Protect pipes through transient control. Opflow, 45(8): 26-28.
Blokker, M., Smeets, P. and Medema, G. (2014). QMRA in the drinking water distribution system. Procedia Eng., 89: 151-159.
Blokker, M., Smeets, P. and Medema, G. (2018). Quantitative microbial risk assessment of repairs of the drinking water distribution system. Microb. Risk Anal., 8: 22-31.
Bokulich, N.A. and Mills, D.A. (2013). Improved selection of internal transcribed spacer-specific primers enables quantitative, ultra-high-throughput profiling of fungal communities. Appl Environ Microbiol., 79(8): 2519-26.
Boulay, N. and Edwards, M. (2001). Role of temperature, chlorine, and organic matter in copper corrosion byproduct release in soft water. Water Res., 35(3): 683-690.
Bourbigot, M.M., Dodin, A. and Lhértier, R. (1982). Limiting bacterial aftergrowth in distribution systems by removing biodegradable organics. Proceedings from the 1982 AWWA Annual Conference and Exhibit. American Water Works Association, Denver, Colorado, 871-886.
Bradley, I.M., Pinto, A.J. and Guest, J.S. (2016). Design and Evaluation of Illumina MiSeq-Compatible, 18S rRNA Gene-Specific Primers for Improved Characterization of Mixed Phototrophic Communities. Appl Environ Microbiol., 82(19): 5878-91.
Bradley, T.C., Haas, C.N. and Sales, C.M. (2020). Nitrification in premise plumbing: A review. Water, 12(3): 830.
Brognaux, A., Han, S., Sørensen, S.J., Lebeau, F., Thonart, P. and Delvigne, F. (2013). A low-cost, multiplexable, automated flow cytometry procedure for the characterization of microbial stress dynamics in bioreactors. Microb. Cell Fact.,12(1).
Brunkard, J.M., Ailes, E., Roberts, V.A., Hill, V., Hilborn, E., Craun, G.F. and Rajasingham, A, Kahler, A., Garrison, L., Hicks, L., Carpenter, J., Wade, T.J., Beach, M.J. and Yoder, J.S. (2011). Surveillance for Waterborne Disease Outbreaks Associated With Drinking Water - United States, 2007-2008. Morb. Mortal. Wkly. Rep., Surveill Summ, 60:12:38-68.
Buchberger, S.G., Carter, J.T., Lee, Y. and Schade, T.G. (2003). Random demands, travel times, and water quality in deadends. AwwaRF, Denver, Colorado.
Bucheli-Witschel, M., Kötzsch, S., Darr, S., Widler, R. and Egli, T. (2012). A new method to assess the influence of migration from polymeric materials on the biostability of drinking water. Water Res., 46(13): 4246-4260.
Burlingame, G.A. Lytle, D.A. and Snoeyink, V.L. (2006). Why red water? Understanding iron release in distribution systems. Opflow, 32(12): 12-16.
Bursill, D. (2001). Drinking water treatment-understanding the processes and meeting the challenges. Water Sci. Technol. Water Supply, 1(1): 1-7.
Camper, A.K. (2004). Involvement of humic substances in regrowth. Int. J. Food Microbiol., 92(3): 355-364.
Camper, A. (2014). Organic matter, pipe materials, disinfectants and biofilms in distribution systems. In: Microbial growth in drinking-water supplies: Problems, causes, control and research needs. van der Kooij, D. and van der Wielen, P.W.J.J. (eds.). IWA Publishing, London, UK, pp. 73-94.
Camper, A.K., Butterfield, P., Ellis, B., Jones, W.L., Anderson, W.B., Huck, P.M., Slawson, R., Volk, C., Welch, N. and LeChevallier, M. (2000). Investigation of the biological stability of water in treatment plants and distribution
systems. Awwa Research Foundation and American Water Works Association, Denver, Colorado.
Canadian Infrastructure Report Card (2016). Informing the Future: The Canadian Infrastructure Report Card. Canadian Construction Association, Canadian Public Works Association, Canadian Society for Civil Engineering and Federation of Canadian Municipalities. Available at: http://canadianinfrastructure.ca/downloads/Canadian_Infrastructure_Report_2016.pdf
Cantor, A.F. (2017). Optimization of phosphorus-based corrosion control chemicals using a comprehensive perspective of water quality. Report #4586. Water Research Foundation, Denver, Colorado.
Cantor, A.F. (2018). Water distribution system monitoring: A practical approach for evaluating drinking water quality. 2nd ed. CRC Press, Boca Raton.
Cantor, A.F. (2021). Insights for a distribution system water sentinel. Virtual Summit AWWA: Lead & Water Quality. April 7-8, 2021. American Water Works Association, Denver, Colorado.
Cantor, A.F. and Cantor, J. (2009). In Dr. Deming's footsteps: Distribution system water quality control and process improvement. Proceedings from the 2009 AWWA water quality technology conference. American Water Works Association (AWWA), Denver, Colorado, 724-741.
Cantor, A.F., Kiefer, E., Little, K.,Jacque, A., Degnan, A., Maynard, B., Mast, D. and Cantor., J. (2012). Distribution system water quality control demonstration. Water Research Foundation, Denver, Colorado.
Carrière, A., Gauthier, V., Desjardins, R., & Barbeau, B. (2005). Evaluation of loose deposits in distribution systems through unidirectional flushing. J. Am. Water Works Assoc., 97(9), 82-92.
CCME (2004). From source to tap: Guidance on the multi-barrier approach to safe drinking water. Canadian Council of Ministers of the Environment, Winnipeg, Manitoba.
Chandy, J.P and Angles, M.L. (2001). Determination of nutrients limiting biofilm formation and the subsequent impact on disinfectant decay. Water Res., 35(11): 2677-2682.
Chang, Y. and Jung, K. (2004). Effect of distribution system materials and water quality on heterotrophic plate counts and biofilm proliferation. J Microbiol Biotechnol, 14(6): 1114-1119.
Chao, Y., Ma, L., Yang, Y., Ju, F., Zhang, X.X., Wu, W.M. and Zhang, T. (2013). Metagenomic analysis reveals significant changes of microbial compositions and protective functions during drinking water treatment. Sci. Rep., 3: 3550.
Chao, Y., Mao, Y., Wang, Z. and Zhang, T. (2015). Diversity and functions of bacterial community in drinking water biofilms revealed by high-throughput sequencing. Sci. Rep., 5: 10044.
Chapman, T. and Ziemann, S. (2019). Watermain monitoring system. Presented at Canadian Water and Wastewater Conference, Banff, Alberta, November 4.
Chowdhury, S. (2012). Heterotrophic bacteria in drinking water distribution system: a review. Environ. Monit. Assess., 184(10): 6087-6137.
Christensen, B.E. and Characklis, W.G. (1990). Physical and chemical properties of biofilms. Chapter 4 in: Biofilm. Characklis, W.G. and Marshall, K.C. (eds). Wiley, New York, 93-130.
Clark, R.M., Yang, Y.J, Impellitteri, C.A., Haught, R.C., Schupp, D.A.S., Panguluri, S. and Krishnan, E.R. (2010). Chlorine fate and transport in distribution systems: Experimental and modelling studies. J. Am. Water Works Assoc., 102(5): 144-155.Codony, F., Morató, J. and Mas, J. (2005). Role of discontinuous chlorination on microbial production by drinking water biofilms. Water Res., 39(9):1896-1906.
Coetser, S. E. and Cloete, T. E. (2005). Biofouling and biocorrosion in industrial water systems. Crit. Rev. Microbiol. 31, 213-232.
Collier, S.A., Deng, L., Adam, E.A., Benedict, K.M., Beshearse, E.M., Blackstock, A.J., Bruce, B.B., Derado, G., Edens, C., Fullerton, K.E., Gargano, J.W., Geissler, A.L., Hall, A.J., Havelaar, A.H., Hill, V.R., Hoekstra, R.M., Reddy, S.C., Scallan, E., Stokes, E.K., Yoder, J.S. and Beach, M.J. (2021). Estimate of burden and direct healthcare cost of infectious waterborne disease in the United States. Emerg Infect Dis., 27(1): 140-149.
Copeland, A. and Lytle, D.A. (2014). Measuring the oxidation-reduction potential of important oxidants in drinking water. J. Am. Water Works Assoc., 106(1): E10-E20.
Córdoba, M.A., Del Coco, V.F., Minvielle, M.C. and Basualdo, J.Á. (2010). Influencing factors in the occurrence of injured coliforms in the drinking water distribution system in the city of La Plata, Argentina. J Water Health, 8(2): 205-211.
Craun, G.F. and Calderon, R.L. (2001). Waterborne disease outbreaks caused by distribution system deficiencies. J. Am. Water Works Assoc., 93(9): 64-75.
Dang, P., Jayaratne, A., and Wilson, G. (2014). Ice pigging - a better way to clean water mains. Yarra Valley Water, Australia.
De Roy, K., Clement, L., Thas, O., Wang, Y. and Boon, N. (2012). Flow cytometry for fast microbial community fingerprinting. Water Res., 46(3): 907-919.
de Vet, W.W.J.M. (2011). Biological drinking water treatment of anaerobic groundwater in trickling filters. Ph. D. dissertation, Technical University Delft, The Netherlands.
Deininger, R.A. and Lee, J. (2001). Rapid determination of bacteria in drinking water using an ATP assay. Field Anal. Chem. Technol., 5(4): 185-189.
Delafont, V., Brouke, A., Bouchon, D., Moulin, L. and Hechard, Y. (2013). Microbiome of free-living amoebae isolated from drinking water. Water Res., 47(19): 6958-6965.
Delahaye E., Welté B., Levi Y., Leblon G. and Montiel A. (2003). An ATP-based method for monitoring the microbiological drinking water quality in a distribution network. Water Res., 37(15): 3689-3696.
Douterelo, I., Boxall, J.B., Deines, P., Sekar, R., Fish, K.E. and Biggs, C.A. (2014a). Methodological approaches for studying the microbial ecology of drinking water distribution systems. Water Res., 65: 134-156.
Douterelo, I., Husband, S. and Boxall, J. B. (2014b). The bacteriological composition of biomass recovered by flushing an operational drinking water distribution system. Water Res 54, 100-114.
Douterelo, I., Husband, S., Loza, V. and Boxall, J. (2016a). Dynamics of biofilm regrowth in drinking water distribution systems. Appl. Environ. Microbiol., 82(14): 4155-4168.
Douterelo, I., Jackson, M., Solomon, C. and Boxall, J. (2016b). Microbial analysis of in situ biofilm formation in drinking water distribution systems: implications for monitoring and control of drinking water quality. Appl Microbiol Biot 100, 3301-3311.
Douterelo, I., Calero-Preciado, C., Soria-Carrasco, V. and Boxall, J.B. (2018). Whole metagenome sequencing of chlorinated drinking water distribution systems. Environ. Sci.: Water Res. Technol., 4: 2080.
Durand, J., Deshommes, E., Nour, S., and Prévost, M. (2016). Monitoring water quality in distribution systems: laboratory and field validation of on-line multi-parameter probes. Paper presented at the 17th Canadian National Conference on Drinking Water, Ottawa, ON.
Ebacher, G., Besner, M-C., Lavoie, J., Jung, B.S. Karney, B.W. and Prévost, M. (2011). Transient modeling of a full-scale distribution system: Comparison with field data. J. Water Resour. Plan. Manag., 137(2): 173-182.
Ebacher, G., Besner, M.C., Clement, B. and Prevost, M. (2012). Sensitivity analysis of some critical factors affecting simulated intrusion volumes during a low pressure transient event in a full-scale water distribution system. Water Res., 46(13): 4017-4030.
Eichler, S., Christen, R., Höltje, C., Westphal, P., Bötel, J., Brettar, I., Mehling, A. and Höfle, M.G. (2006). Composition and dynamics of bacterial communities of a drinking water supply system as assessed by RNA- and DNA-based 16S rRNA gene fingerprinting. Appl. Environ. Microbiol., 72(3): 1858-1872.
El-Chakhtoura, J., Prest, E., Saikaly, P., van Loosdrecht, M., Hammes, F. and Vrouwenvelder, H. (2015). Dynamics of bacterial communities before and after distribution in a full-scale drinking water network. Water Res., 74: 180-90.
Ellison, D., Duranceau, S.J., Ancel, S. Deagle, G. and McCoy, R. (2003). Investigation of pipe cleaning methods. AwwaRF, Denver, Colorado.
Emde, K.M.E., Smith, D.W. and Facey, R. (1992). Initial investigation of microbially influenced corrosion (MIC) in a low temperature water distribution system. Water Res., 26(2): 169-175.
Ercumen, A., Gruber, J.S. and Colford, J.M. Jr. (2014). Water distribution system deficiencies and gastrointestinal illness: a systematic review and meta-analysis. Environ Health Perspect., 122(7):651-60.
Escobar, I.C. and Randall, A.A. (2001). Assimilable organic carbon (AOC) and biodegradable dissolved organic carbon (BDOC): Complementary measurements. Water Res., 35(18): 4444-4454.
Falkinham, J.O., Pruden, A. and Edwards, M. (2015). Opportunistic premise plumbing pathogens: Increasingly important pathogens in drinking water. Pathogens, 4(2): 373-386.
Favere, J., Buysschaert, B., Boon, N. and De Gusseme, B. (2020). Online microbial fingerprinting for quality management of drinking water: Full-scale event detection. Water Res., 170: 115353.
Feazel, L.M., Baumgartner, L.K., Peterson, K.L., Frank, D.N., Harris, J.K. and Pace, N.R. (2009). Opportunistic pathogens enriched in showerhead biofilms. Proc Natl Acad Sci USA, 106(38): 16393-9.
Fish, K.E., Osborn, A.M. and Boxall, J. (2016). Characterising and understanding the impact of microbial biofilms and the extracellular polymeric substance (EPS) matrix in drinking water distribution systems. Environ. Sci. Water Res. Technol., 2 (4): 614-630.
Fitchett, J.C., Karadimitriou, K., West, Z. and Hughes, D.M. (2020). Machine learning for pipe condution assessments. J. Am. Water Works Assoc., 112(5): 50-55.
Flemming, H-C. and Wingender, J. (2001). Relevance of microbial extracellular polymeric substances (EPSs) – Part I: Structural and ecological aspects. Water Sci Technol. 43(6):1-8.
Flemming, H. and Wingender, J. (2010). The biofilm matrix. Nat. Rev. Microbiol., 8(9): 623-633.
Flemming, H., Percival, S.L. and Walker, J.T. (2002). Contamination potential of biofilms in water distribution systems. Water Sc. Technol. Water Supply, 2(1): 271-280.
Fontanazza, C.M., Notaro, V., Puleo, V., Nicolosi, P. and Freni, G. (2015). Contaminant intrusion through leaks in water distribution system: Experimental analysis. Procedia Eng., 119(1): 426-433.
Fox, S., Shepherd, W., Collins, R., and Boxall., J. (2016). Experimental quantification of contaminant ingress into a buried leaking pipe during transient events. J. Hydraul. Eng., 142(1): 04015036.
Frey, M.M. and Sullivan, L. (2004). Practical application of online monitoring. Awwa Research Foundation, Denver, Colorado.
Friedman, M. (2014). Use of monitoring, bench-scale and pipe rig studies to solve a pipe destabilization mystery. City of Longview, WA. In: Proceedings of the American Water Works Association Water Quality Technology Conference, New Orleans, Louisiana. American Water Works Association, Denver, Colorado.
Friedman, M. (2020). Planning for source water and treatment changes. Presented at the US EPA 17th annual drinking water workshop: Small systems challenges and solutions. September 2.
Friedman, M. and Slabaugh, R. (2020). Selecting and implementing CCT. Presented at the US EPA 17th annual drinking water workshop: Small systems challenges and solutions. August 31.
Friedman, M., Martel, K. Hill, A., Holt, D., Smith, S., Ta, T., Sherwin, C., Hiltebrand, D., Pommerenk, P., Hinedi, Z. and Camper, A. (2003). Establishing site-specific flushing velocities. Awwa Research Foundation, Denver, Colorado and Kiwa, N.V., Nieuwegein, The Netherlands.
Friedman, M., Radder, L., Harrison, S., Howie, D., Britton, M. Boyd, G., Wang, H., Gullick, R., LeChevallier, M., Wood, D. and Funk, J. (2004). Verification and control of pressure transients and intrusion in distribution systems. Awwa Research Foundation, Denver, Colorado and US Environmental Protection Agency, Washington, DC.
Friedman, M.J., Hill, A.S., Reiber, S.H., Valentine, R.L. and Korshin, G.V. (2010a). Assessment of inorganics accumulation in drinking water system scales and sediments. Water Research Foundation, Denver, Colorado.
Friedman, M., Kirmeyer, G., Lemieux, J., LeChevalllier, M., Seidl, S. and Routt, J. (2010b). Criteria for optimized distribution systems. Water Research Foundation, Denver, Colorado.
Friedman, M., Hill, A., Booth, S., Hallett, M., McNeill, L., McLean, J., Sorensen, D., Hammer, T., De Haan, M., MacArthur, K. and Mitchell, K. (2016). Metals accumulation and release within the distribution system: Evaluation and mitigation. Report #4509. Water Research Foundation, Denver, Colorado.
Gagnon, G.A., Murphy, H.M., Rand, J.L., Payne, S.J., Springthorpe, S., Matias, F., Nokbeh, R. and Sattar, S.S. (2007). Coliforms in distribution systems; integrated disinfection and antimicrobial resistance. AWWA, AWWA Research Foundation, IWA, Denver, Colorado.
Gagnon, G.A., Baribeau, H., Rutledge, S.O., Dumancic, R., Oehmen, A., Chauret, C. and Andrews, S. (2008). Disinfectant efficacy in distribution systems: a pilot-scale assessment. J. Water Supply Res. Technol. AQUA, 57(7): 507-518.
Garner, E., Davis, B.C., Milligan, E., Blair, M.F., Keenum, I., Maile-Moskowitz, A., Pan, J., Gnegy, M., Liguori, K., Gupta, S., Prussin, J. 2nd, Marr, L.C., Heath, L.S., Vikesland, P.J., Zhang, L. and Pruden A. (2021). Next generation sequencing approaches to evaluate water and wastewater quality. Water Res., 194: 116907.
Gauthier, V., Gérard, B., Portal, J-M., Block, J.C., and Gatel, D. (1999). Organic matter as loose deposits in a drinking water distribution system. Water Res. 33, 1014-1026.
Gibbons, C.L., Mangen, M.-.J., Plass, D., Havelaar, A.H., Brooke, R.J., Kramarz, P., Peterson, K.L., Stuurman, A.L., Cassini, A., Fèvre, E.M. and Kretzschmar, M.E. (2014). Measuring underreporting and under-ascertainment in infectious disease datasets: A comparison of methods. BMC Public Health, 14(1).
Gillespie, S., Lipphaus, P., Green, J., Parsons, S., Weir, P., Juskowiak, K., Jefferson, B., Jarvis, P. and Nocker, A. (2014). Assessing microbiological water quality in drinking water distribution systems with disinfectant residual using flow cytometry. Water Res., 65: 224-234.
Ginn, T. and Smither, B. (2020). Pressure-transient monitoring supports asset management. Opflow, 46(12): 28-30.
GLUMRB (2012). Recommended standards for water works. Health Education Services, Great Lakes - Upper Mississippi River Board of State and Provincial Public Health and Environmental Managers, Albany, New York.
Gomez-Alvarez, V., Revetta, R.P. and Santo Domingo, J.W. (2012). Metagenomic analyses of drinking water receiving different disinfection treatments. Appl. Environ. Microbiol., 78(17): 6095-6102.
Gomez-Alvarez, V., Humrighouse, B.W., Revetta, R.P. and Santo Domingo, J.W. (2015). Bacterial composition in a metropolitan drinking water distribution system utilizing different source waters. J. Water Health, 13(1): 140-151.
Goncharuk, V.V., Bagrii, V.A., Mel'nik, L.A., Chebotareva, R.D. and Bashtan, S.Y. (2010). The use of redox potential in water treatment processes. J. Water Chem. Technol. 32(1): 1-9.
Goordial, J., Altshuler, I., Hindson, K., Chan-Yam, K., Marcolefas, E. and Whyte, L.G. (2017). In situ field sequencing and life detection in remote (79◦26′N) Canadian high arctic permafrost ice wedge microbial communities. Front Microbiol, 8: 2594.
Gullick, R.W., LeChevallier, M.W., Svindland, R.C. and Friedman, M.J. (2004). Occurrence of transient low and negative pressures in distribution systems. J. Am. Water Works Assoc., 96(11): 52-66.
Haas, C.N. (1999). Benefits of using a disinfectant residual. J. Am. Water Works Assoc., 91(1): 65-69.
Halton (2001). Investigation of material deposits in the Burlington distribution system. Regional Municipality of Halton, Oakville, Ontario.
Hamilton, D. and Kikolica, P. (2021) The City of Hamilton reduces non-revenue water loss with proactive leak detection program. Ontario Pipeline, Spring 2021.
Hammes, F.A. and Egli, T. (2005). New method for assimilable organic carbon determination using flow-cytometric enumeration and a natural microbial consortium as inoculum. Environ. Sci. Technol., 39(9): 3289-3294.
Hammes, F. and Egli, T. (2010). Cytometric methods for measuring bacteria in water: Advantages, pitfalls and applications. Anal. Bioanal Chem., 397(3): 1083-1095.
Hammes, F., Berney, M., Wang, Y., Vital, M., Koster, O. and Egli, T. (2008). Flow-cytometric total bacterial cell counts as a descriptive microbiological parameter for drinking water treatment processes. Water Res., 42(1-2): 269-277.
Hammes, F., Goldschmidt, F., Vital, M., Wang, Y. and Egli, T. (2010). Measurement and interpretation of microbial adenosine tri-phosphate (ATP) in aquatic environments. Water Res., 44(13): 3915-3923.
Hammes, F., Boon, N., Vital, M., Ross, P., Magic-Knezev, A. and Dignum, M. (2011). Bacterial colonization of pellet softening reactors used during drinking water treatment. Appl. Environ. Microbiol., 77(3): 1041-1048.
Hammes, F., Broger, T., Weilenmann, H.U., Vital, M., Helbing, J., Bosshart, U., Huber, P., Odermatt, R.P. and Sonnleitner, B. (2012). Development and laboratory-scale testing of a fully automated online flow cytometer for drinking water analysis. Cytometry A., 81(6): 508-516.
Hargesheimer, E. (2001). Walkerton Inquiry Part II: review of issue #7 : Measurement of source and finished water quality. Prepared on behalf of the Ontario Water Works Association and the Ontario Municipal Water Association. Available at: http://www.archives.gov.on.ca/en/e_records/walkerton/part2info/partieswithstanding/pdf/OWWA7.pdf.
Hatam, F., Besner, M-C., Ebacher, G. and Prévost, M. (2020). Limitations of E. coli monitoring for confirmation of contamination in distribution systems due to intrusion under low pressure condition in the presence of disinfectants. J. Water Resour. Plann. Manage. 146(8): 04020056.
Health Canada (2009a) Guidance on controlling corrosion in drinking water distribution systems. Water, Air and Climate Change Bureau, Healthy Environments and Consumer Safety Branch, Health Canada, Ottawa, Ontario. Available at: www.canada.ca/en/health-canada/services/publications/healthy-living/guidance-controlling-corrosion-drinking-water-distribution-systems.html
Health Canada (2009b) Guidelines for Canadian Drinking Water Quality: Guideline Technical Document — Chlorine. Water, Air and Climate Change Bureau, Healthy Environments and Consumer Safety Branch, Health Canada, Ottawa, Ontario. Available at: www.canada.ca/en/health-canada/services/publications/healthy-living/guidelines-canadian-drinking-water-quality-chlorine-guideline-technical-document.html
Health Canada (2012). Guidance on the use of heterotrophic plate counts in Canadian drinking water supplies. Water, Air and Climate Change Bureau, Healthy Environments and Consumer Safety Branch, Health Canada, Ottawa, Ontario. Available at: www.canada.ca/en/health-canada/services/publications/healthy-living/guidance-use-heterotrophic-plate-counts-canadian-drinking-water-supplies.html
Health Canada (2013). Guidance on Providing Safe Drinking Water in Areas of Federal Jurisdiction. Version 2, Water and Air Quality Bureau, Healthy Environments and Consumer Safety Branch, Health Canada, Ottawa, Ontario. Available at: www.canada.ca/en/health-canada/services/publications/healthy-living/guidance-providing-safe-drinking-water-areas-federal-jurisdiction-version-2.html
Health Canada (2015). Guidelines for Canadian Drinking Water Quality: Guideline Technical Document — pH. Water and Air Quality Bureau, Healthy Environments and Consumer Safety Branch, Health Canada, Ottawa, Ontario. Available at: www.canada.ca/en/health-canada/services/publications/healthy-living/guidelines-canadian-drinking-water-quality-guideline-technical-document-ph.html
Health Canada (2019). Personal communication with T. Beattie. Analysis based on data from the Drinking Water Application (DWA) of the Canadian Network for Public Health Intelligence (CNPHI).
Health Canada (2020a). Guidelines for Canadian drinking water quality: Guideline technical document — Escherichia coli. Water and Air Quality Bureau, Healthy Environments and Consumer Safety Branch, Health Canada, Ottawa, Ontario. Available at: www.canada.ca/en/health-canada/services/publications/healthy-living/guidelines-canadian-drinking-water-quality-guideline-technical-document-escherichia-coli.html
Health Canada (2020b). Guidelines for Canadian drinking water quality: Guideline technical document — total coliforms. Water and Air Quality Bureau, Healthy Environments and Consumer Safety Branch, Health Canada, Ottawa, Ontario. Available at: www.canada.ca/en/health-canada/services/publications/healthy-living/guidelines-canadian-drinking-water-quality-guideline-technical-document-total-coliforms.html
Health Canada (2020c). Guidance on natural organic matter in drinking water. Water Quality and Health Bureau, Healthy Environments and Consumer Safety Branch, Health Canada, Ottawa, Ontario. Available at: www.canada.ca/en/health-canada/services/publications/healthy-living/guidance-natural-organic-matter-drinking-water.html
Health Canada (2021a). Guidance on waterborne pathogens - consultation document. Water and Air Quality Bureau, Healthy Environments and Consumer Safety Branch, Health Canada, Ottawa, Ontario. Available at: www.canada.ca/en/health-canada/programs/consultation-proposed-guidance-waterborne-pathogens/document.html
Health Canada (2021b) Guidance on the Temperature Aspects of Drinking Water. Water, Air and Climate Change Bureau, Healthy Environments and Consumer Safety Branch, Health Canada, Ottawa, Ontario. Available at: https://www.canada.ca/en/health-canada/services/publications/healthy-living/guidelines-canadian-drinking-water-quality-guideline-technical-document-temperature.html
Health Canada (2021c). Guidelines for Canadian drinking water quality: Guideline technical document — aluminum. Water and Air Quality Bureau, Healthy Environments and Consumer Safety Branch, Health Canada, Ottawa, Ontario. Available at: www.canada.ca/en/health-canada/services/environmental-workplace-health/reports- publications/water-quality.html
Henne, K., Kahlisch, L., Brettar, I. and Höfle, M.G. (2012). Analysis of structure and composition of bacterial core communities in mature drinking water biofilms and bulk water of a citywide network in Germany. Appl. Environ. Microbiol., 78(10): 3530-3538.
Hilborn, E. D., Wade, T., Hicks, L., Garrison, L. and Gargano, J. (2013). Surveillance for waterborne disease outbreaks associated with drinking water and other nonrecreational water - United States, 2009-2010. Morb. Mortal. Wkly. Rep., 62(35): 714-720.
Hill, A.S., Friedman, M., Hallett, M., Salo-Zieman, V., Booth, S., Hanson, A., Gupta, K., Akagi, Y., Kochiss, C., Koperski, L., Igoe, P., Kirby, L. and Harper, W. (2018). Use of flushing as a corrective action under the revised total coliform rule. Report #4653. Water Research Foundation, Denver, Colorado.
Hooper, J., Vickstrom, K., Evans, P., Alito, C., Black, S. and Lauderdale, C. (2019). Guidance manual for monitoring biological filtration of drinking water. Report #4620. Water Research Foundation, Denver, Colorado.
Hong, P., Hwang, C., Ling, F., Andersen, G.L., LeChevallier, M.W. and Liu, W. (2010). Pyrosequencing analysis of bacterial biofilm communities in water meters of a drinking water distribution system. Appl. Environ. Microbiol., 76(16): 5631-5635.
Howe, A.D., Forster, S., Morton, S., Marshall, R., Osborn, K.S., Wright, P. and Hunter, P.R. (2002). Cryptosporidium oocysts in a water supply associated with a cryptosporidiosis outbreak. Emerg. Infect. Dis., 8(6): 619-624.
Hrudey, S.E. and Hrudey, E.J. (2004). Safe drinking water: Lessons from recent outbreaks in affluent nations. IWA Publishing, London, England.
Hrudey, S.E. and Hrudey, E.J. (2014). Ensuring safe drinking water: Learning from frontline experience with contamination. American Water Works Association, Denver, Colorado.
Huck, P. M. (1990). Measurement of biodegradable organic matter and bacterial growth potential in drinking water. J. Am. Water Works Assoc., 82: 78-86.
Hunter, P.R., Chalmers, R.M., Hughes, S. and Syed, Q. (2005). Self-reported diarrhea in a control group: A strong association with reporting of low-pressure events in tap water. Clin. Infect. Dis., 40(4): e32-34.
Husband, S. and Boxall, J.B. (2010). Field studies of discoloration in water distribution systems: Model verification and practical implications. J. Environ. Eng., 136(1): 86-94.
Husband, S., Fish, K.E., Douterelo, I. and Boxall, J. (2016). Linking discolouration modelling and biofilm behaviour within drinking water distribution systems. pp. 942-950.
Hwang, C., Ling, F., Andersen, G.L., LeChevallier, M.W. and Liu, W. (2011). Evaluation of methods for the extraction of DNA from drinking water distribution system biofilms. Microbes and Environments, 27(1): 9-18.
Hwang, C., Ling, F., Andersen, G.L., LeChevallier, M.W. and Liu, W. (2012). Microbial community dynamics of an urban drinking water distribution system subjected to phases of chloramination and chlorination treatments. Appl. Environ. Microbiol., 78(22): 7856-7865.
Islam, N., Al-Zahrani, M.A.M., Sadiq, R., Farahat, A. and Rodriguez, M.J. (2015). Contaminant intrusion in water distribution networks: Review and proposal of an integrated model for decision making. Env. Rev., 23(3): 337-352.
Jakopanec, I., Borgen, K., Vold, L., Lund, H., Forseth, T., Hannula, R. and Nygård, K. (2008). A large waterborne outbreak of Campylobacteriosis in Norway: The need to focus on distribution system safety. BMC Infect. Dis., 8.
Kachani, Y., Laneuville, M. and Jernigan, W. (2020). Developing Quebec's water efficiency strategy. J. Am. Water Works Assoc., 112(6): 56-64.
Karim, M.R., Abbaszadegan, M. and Lechevallier, M. (2003). Potential for pathogen intrusion during pressure transients. J. Am. Water Works Assoc., 95(5): 134-146.
Kennedy, L.C., Miller, S.E., Kantor, R.S. and Nelson, K.L. (2021). Effect of disinfectant residual, pH, and temperature on microbial abundance in disinfected drinking water distribution systems. Environ. Sci.: Water Res. Technol., 7: 78-92
Kirmeyer, G.J., Kirby, L., Murphy, B.M., Noran, P.F., Martel, K.D., Lund, T.W., Anderson, J.L. and Medhurst, R. (1999). Maintaining water quality in finished water storage facilities. Awwa Research Foundation and American Water Works Association, Denver, Colorado.
Kirmeyer, G.J., Friedman, M., Clement, J., Sandvig, A., Noran, P.F, Martel, K.D., Smith, D., LeChevallier, M.W., Volk, C., Antoun, E., Hiltebrand, D., Dyksen, J. and Cushing. R.S. (2000). Guidance manual for maintaining distribution system water quality. Awwa Research Foundation and American Water Works Association, Denver, Colorado.
Kirmeyer, G.J., Friedman, M., Martel, K., Howie, D., LeChevallier, M., Abbaszadegan, M., Karim, M., Funk, J. and Harbour, J. (2001). Pathogen intrusion into the distribution system. AWWA Research Foundation and American Water Works Association, Denver, Colorado.
Kirmeyer, G.J., Thomure, T.M., Rahman, R., Marie, J.L., LeChevallier, M.W., Yang, J., Hughes, D.M. and Schneider, O. (2014). Effective microbial control strategies for main breaks and depressurization. Water Research Foundation, Denver, Colorado.
Koch, C., Harnisch, F., Schröder, U. and Müller, S. (2014). Cytometric fingerprints: Evaluation of new tools for analyzing microbial community dynamics. Front. Microbiol., 5.
Laine, J., Huovinen, E., Virtanen, M.J., Snellman, M., Lumio, J., Ruutu, P., Kujansuu, E., Vuento, R., Pitkänen, T., Miettinen, I., Herrala, J., Lepistö, O., Antonen, J., Helenius, J., Hänninen, M., Maunula, L., Mustonen, J., Kuusi, M., Collin, P., Korpela, M., Kuusela, A., Mustajoki, S., Oksa, H., Räsänen, S., Uotila, T. and Katto, T. (2011). An extensive gastroenteritis outbreak after drinking-water contamination by sewage effluent, Finland. Epidemiol. Infect., 139(7): 1105-1113.
Lambertini, E., Borchardt, M.A., Kieke, B.A., Spencer, S.K. and Loge, F.J. (2012). Risk of viral acute gastrointestinal illness from nondisinfected drinking water distribution systems. Environ. Sci. Technol., 46(17): 9299-9307.
Lan, Y., Rosen, G. and Hershberg, R. (2016). Marker genes that are less conserved in their sequences are useful for predicting genome-wide similarity levels between closely related prokaryotic strains. Microbiome, 4(1): 18.
Lautenschlager, K., Boon, N., Wang, Y., Egli, T. and Hammes, F. (2010). Overnight stagnation of drinking water in household taps induces microbial growth and changes in community composition. Water Res., 44(17): 4868-4877.
Lautenschlager, K., Hwang, C., Liu, W.T., Boon, N., Koster, O., Vrouwenvelder, H., Egli, T. and Hammes, F. (2013). A microbiology-based multi-parametric approach towards assessing biological stability in drinking water distribution networks. Water Res., 47(9): 3015-3025.
LeChevallier, M.W. (1999). Biofilms in drinking water distribution systems: Significance and control. In: Identifying future drinking water contaminants: 1998 workshop on emerging drinking water contaminants. National Research Council (ed.). The National Academies Press, Washington, DC, pp. 206-219.
LeChevallier, M.W. and Au, K-K. (2004). Water treatment and pathogen control. Process efficiency in achieving safe drinking water. World Health Organization, 2004. IWA Publishing. London.
LeChevallier, M.W., Cawthorn, C.D. and Lee, R.G. (1988). Mechanisms of bacterial survival in chlorinated drinking water. Water Sci. Technol., 20(11-12): 145-151.
LeChevallier, M.W., Olson, B.H. and McFeters, G.A. (1990). Assessing and controlling bacterial regrowth in distribution systems. Awwa Research Foundation, Denver, Colorado.
LeChevallier, M.W., Welch, N.J. and Smith, D.B. (1996). Full-scale studies of factors related to coliform regrowth in drinking water. Applied and Environmental Microbiology, 62 (7), pp. 2201-2211.
LeChevallier, M.W., Gullick, R.W., Karim, M.R., Friedman, M. and Funk, J.E. (2003). The potential for health risks from intrusion of contaminants into the distribution system from pressure transients. J. Water Health, 1(1): 3-14.
LeChevallier, M. W., M. Xu, J. Yang, P. Teunis, and Fleming, K. (2011). Managing distribution system low transient pressures for water quality. Water Research Foundation, Denver, Colorado.
LeChevallier, M. W., M. Xu, J. Yang, P. Teunis, and Fleming, K. (2014). Pressure management: Industry practices and monitoring procedures. Water Research Foundation, Denver, Colorado.
LeChevallier, M.W., Schneider, O.D., Weinrich, L.A., Jjemba, P.K., Evans, P.J., Hooper, J.L. and Chappell, R.W. (2015a). An operational definition of biostability in drinking water. Water Research Foundation, Denver, Colorado.
LeChevallier, M.W., Schneider, O.D., Weinrich, L.A., Jjemba, P.K., Evans, P.J., Hooper, J.L. and Chappell, R.W. (2015b). Guidance manual for control of biostability in drinking water. Water Research Foundation, Denver, Colorado.
Lee, Y. (2013). Factors controlling bacterial growth in drinking water distribution pipes. Asian J Chem, 25(3): 1629-1634.
Lee, S.H., Levy, D.A., Craun, G.F., Beach, M.J. and Calderon, R.L. (2002). Surveillance for waterborne-disease outbreaks - United States, 1999-2000. MMWR Surveill Summ, 51(8): 1-47.
Lee, J.J., Schwartz, P., Sylvester, P., Crane, L., Haw, J., Chang, H. and Kwon, H.J. (2003). Impacts of cross-connections in North American water supplies. Water Research Foundation, Denver, Colorado.Lehtola, M. J.,
Levin, R.B., Epstein, P.R., Ford, T.E., Harrington, W., Olson, E. and Reichard, E.G. (2002). US drinking water challenges in the twenty-first century. Environ. Health Perspect., 110: 43-52.
Levy, D.A., Bens, M.S., Craun, G.F., Calderon, R.L. and Herwaldt, B.L. (1998). Surveillance for Waterborne-Disease Outbreaks - United States 1995-1996. Morb. Mortal. Wkly. Rep., 4
Li, G., Ding, Y., Xu, H., Jin, J. and Shi, B. (2018). Characterization and release profile of (Mn, Al)-bearing deposits in drinking water distribution systems. Chemosphere, 197: 73-80.
Liang, J.L., Dziuban, E.J., Craun, G.F., Hill, V., Moore, M.R., Gelting, R.J., Calderon, R.L., Beach, M.J. and Roy, S.L. (2006). Surveillance for waterborne disease and outbreaks associated with drinking water and water not intended for drinking - United States, 2003-2004. MMWR Surveill Summ, 55(12): 31-65.
Lillis, T.O. and Bissonnette, G.K. (2001). Detection and characterization of filterable heterotrophic bacteria from rural groundwater supplies. Lett. Appl. Microbiol., 32(4): 268-272.
Lindley, T.R. and Buchberger, S.G. (2002). Assessing intrusion susceptibility in distribution systems. J. Am. Water Works Assoc., 94(6): 66-79.
Ling, F., Hwang, C., LeChevallier, M.W., Andersen, G.L. and Liu, W-T. (2016). Core-satellite populations and seasonality of water meter biofilms in a metropolitan drinking water distribution system. ISME Journal, 10(3): 582-595.
Lipphaus, P., Hammes, F., Kötzsch, S., Green, J., Gillespie, S. and Nocker, A. (2014). Microbiological tap water profile of a medium-sized building and effect of water stagnation. Environmental Technology (United Kingdom), 35(5): 620-628.
Liu, G., Verberk, J.Q.J.C. and Van Dijk, J.C. (2013a). Bacteriology of drinking water distribution systems: An integral and multidimensional review. Appl Microbiol Biotechnol., 97: 9265-9276.
Liu, G., Lut, M.C., Verberk, J.Q.J.C. and Van Dijk, J.C. (2013b). A comparison of additional treatment processes to limit particle accumulation and microbial growth during drinking water distribution. Water Res., 47(8): 2719-2728.
Liu, G., Ling, F.Q., Magic-Knezev, A., Liu, W.T., Verberk, J.Q.J.C. and Van Dijk, J.C. (2013c). Quantification and identification of particle-associated bacteria in unchlorinated drinking water from three treatment plants by cultivation-independent methods. Water Res., 47(10): 3523-3533.
Liu, G., Bakker, G.L., Li, S., Vreeburg, J.H.G., Verberk, J.Q.J.C., Medema, G.J., Liu, W.T. and Van Dijk, J.C. (2014). Pyrosequencing reveals bacterial communities in unchlorinated drinking water distribution system: An integral study of bulk water, suspended solids, loose deposits, and pipe wall biofilm. Environ. Sci.Technol., 48(10): 5467-5476.
Liu, G., Liu, W., Zhang, Y., Knibbe, W., Feng, C., Medema, G. and van der Meer, W. (2017). Potential impacts of changing supply-water quality on drinking water distribution: A review. Water Res., 116: 135-148.
Liu, G., Zhang, Y., van der Mark, E., Magic-Knezev, A., Pinto, A., van den Bogert, B., Liu, W., van der Meer, W. and Medema, G. (2018). Assessing the origin of bacteria in tap water and distribution system in an unchlorinated drinking water system by SourceTracker using microbial community fingerprints. Water Res., 138: 86-96.
Liu, S., Gunawan, C., Barraud, N., Rice, S.A., Harry, E.J. and Amal, R. (2016). Understanding, monitoring, and controlling biofilm growth in drinking water distribution systems. Environ. Sci. Technol., 50(17): 8954-8976.
Locco, D. and Alberton, D. (2021) Hamilton conducts dead-end watermain flushing program using post hydrants. Envrionmental Science & Engineering, February 2021.
Locco, D., Waller, M., Giani, R., Nikolica, P., Hill, A. and Friedman, M. (2018). Pilot testing for optimized unidirectional flushing in the Hamilton distribution system. In: Proceedings of the American Water Works Association Annual Conference and Exposition, Las Vegas, Nevada. American Water Works Association, Denver, Colorado.
Lotimer, T. (2012). The role of well integrity in reducing pathogen contamination of public groundwater supplies. Canadian Water Network Conference: Assessing pathogen fate, transport and risk in natural and engineered water treatment. Banff, Alberta, Canada, September 23-26, 2012.
Lu, J., Struewing, I., Yelton, S. and Ashbolt, N. (2015). Molecular survey of occurrence and quantity of Legionella spp., Mycobacterium spp., Pseudomonas aeruginosa and amoeba hosts in municipal drinking water storage tanks. J. Appl. Microbiol. 119(1): 278-288.
MacDonald, J. (2001). Walkerton Inquiry Part II: review of issue #8: Production and distribution of drinking water. Prepared on behalf of the Ontario Water Works Association and the Ontario Municipal Water Association. Available at : http://www.ontla.on.ca/library/repository/mon/2000/10297945.pdf
MacDougall, L., Majowicz, S., Doré, K., Flint, J., Thomas, K., Kovacs, S. and Sockett, P. (2008). Under-reporting of infectious gastrointestinal illness in British Columbia, Canada: Who is counted in provincial communicable disease statistics? Epidemiol. Infect., 136(2): 248-256.
Majowicz, S.E., Doré, K., Flint, J.A., Edge, V.L., Read, S., Buffett, M.C., McEwen, S., McNab, W.B., Stacey, D., Sockett, P. and Wilson, J.B. (2004). Magnitude and distribution of acute, self-reported gastrointestinal illness in a Canadian community. Epidemiol. Infect., 132(4): 607-617.
Manuel, C.M., Nunes, O.C. and Melo, L.F. (2010). Unsteady state flow and stagnation in distribution systems affect the biological stability of drinking water. Biofouling, 26(2): 129-139.
Martel, K.D., Kirmeyer, G.J., Murphy, B.M., Noran, P.F., Kirby, L., Lund, T.W., Anderson, J.L., Medhurst, R. and Caprara, M. (2002). Preventing water quality deterioration in finished water storage facilities. J. Am. Water Works Assoc., 94(4): 139-148.
McIlwain, B. (2020). Microbial monitoring in drinking water distribution systems using ATP. Proceedings of AWWA webinar: Investigating ATP analysis for infrastructure release for service. American Water Works Association, Denver, Colorado.
McKinnon, K.M. (2018). Flow cytometry: an overview. Curr Protoc Immunol., 120: 5.1.1-5.1.11.
Messner, M., Shaw, S., Regli, S., Rotert, K., Blank, V. and Soller, J. (2006). An approach for developing a national estimate of waterborne disease due to drinking water and a national estimate model application. J Water Health, 4(SUPPL. 2): 201-240.
Meteer, L. (2018). The distribution trifecta: theory, measurement and impacts to drinking water. York Region.
Moe, C.L and Rheingans, R.D. (2006). Global challenges in water, sanitation and health. J Water Health, 4 (Suppl 1): 41-57.
Moreira, N.A. and Bondelind, M. (2017). Safe drinking water and waterborne outbreaks. J Water Health, 15(1): 83-96.
Mosse, P. and Murray, B. (2015). Good practice guide to the operation of drinking water supply systems for the management of microbial risk. Water for the wellbeing of all Australians. Water Research Australia. Project 1074.
Murphy, H.M., Thomas, M.K., Medeiros, D.T., McFadyen, S. and Pintar, K.D. (2016). Estimating the number of cases of acute gastrointestinal illness (AGI) associated with Canadian municipal drinking water systems. Epidemiol. Infect., 144(7): 1371-1385.
Muylwyk, Q. and MacDonald, J. (2001). Aluminum deposits in the distribution system: What can you do? In: Proceedings of the American Water Works Association Water Quality Technology Conference, Nashville, Tennessee. American Water Works Association, Denver, Colorado.
Najm, I.N., Bouls, L., LeChevallier, M., Norton, C., Volk, C., Randall, A., Escobar, I., Klene, L. and Campos, C. (2000). Case studies of the impacts of treatment changes on biostability in full scale distribution systems. Awwa Research Foundation and American Water Works Association, Denver, Colorado.
NAS. (2019). Management of Legionella in water systems. National Academies of Sciences, Engineering, and Medicine. Washington, DC. The National Academies Press.
Nescerecka, A., Rubulis, J., Vital, M., Juhna, T. and Hammes, F. (2014). Biological instability in a chlorinated drinking water distribution network. PLoS One, 9(5): e96354.
Nescerecka, A., Juhna, T. and Hammes, F. (2016). Behavior and stability of adenosine triphosphate (ATP) during chlorine disinfection. Water Res., 101: 490-497.
Nescerecka, A., Juhna, T. and Hammes, F. (2018). Identifying the underlying causes of biological instability in a full-scale drinking water supply system. Water Res., 135: 11-21.
Nielsen, P.H., Jahn, A. and Palmgren, R. (1997). Conceptual model for production and composition of exopolymers in biofilms. Water Sci. Technol., 36(1): 11-19.
Niquette, P., Servais, P. and Savoir, R. (2000). Impacts of pipe materials on densities of fixed bacterial biomass in a drinking water distribution system. Water Res., 34(6): 1952-1956.
Nocker, A., Sossa, K.E. and Camper, A.K. (2007). Molecular monitoring of disinfection efficacy using propidium monoazide in combination with quantitative PCR. J. Microbiol. Methods, 70(2): 252-260.
Nocker, A., Cheswick, R., Dutheil de la Rochere, P.M., Denis, M., Leziart, T. and Jarvis, P. (2017). When are bacteria dead? A step towards interpreting flow cytometry profiles after chlorine disinfection and membrane integrity staining. Environ. Technol., 38(7): 891-900.
Norton, C.D. and LeChevallier, M.W. (2000). A pilot study of bacteriological population changes through potable water treatment and distribution. Appl. Environ. Microbiol., 66(1): 268-276.
Norton, C.D., LeChevallier, M.W. and Falkinham, J.O. III. (2004). Survival of Mycobacterium avium in a model distribution system. Water Res., 38: 1457-1466.
NRC (2006). Drinking water distribution systems: Assessing and reducing risks. National Research Council. The National Academies Press, Washington, DC.
Nygård, K., Vold, L., Halvorsen, E., Bringeland, E., Røttingen, J.A. and Aavitsland, P. (2004). Waterborne outbreak of gastroenteritis in a religious summer camp in Norway, 2002. Epidemiol. Infect., 132(2): 223-229.
Nygård, K., Wahl, E., Krogh, T., Tveit, O.A., Bøhleng, E., Tverdal, A. and Aavitsland, P. (2007). Breaks and maintenance work in the water distribution systems and gastrointestinal illness: A cohort study. Int. J. Epidemiol., 36(4): 873-880.
Ochromowicz, K. and Hoekstra, E.J. (2005). ATP as an indicator of microbiological activity in tap water. European Commission, DG Joint Research Centre. Report no. EUR 22157 EN.
O'Connor, D.R. (2002). Report of the Walkerton inquiry, Part Two: a strategy for safe drinking water. The Walkerton Inquiry. Toronto, ON. Available at: http://www.archives.gov.on.ca/en/e_records/walkerton/report2/index.html
Park, S.R., Mackay, W.G. and Reid, D.C. (2001). Helicobacter sp. recovered from drinking water biofilm sampled from a water distribution system. Water Res., 35(6): 1624-1626.
Payment, P., Richardson, L., Siemiatycki, J., Dewar, R., Edwardes, M., and Franco, E. (1991). A randomized trial to evaluate the risk of gastrointestinal disease due to consumption of drinking water meeting current microbiological standards. Am J Public Health, 81(6): 703-8.
Payment, P., Siemiatycki, J., Richardson, L., Renaud, G., Franco, E. and Prévost, M. (1997). A prospective epidemiological study of gastrointestinal health effects due to the consumption of drinking water. Int. J. Environ. Health Res., 7(1): 5-31.
Pepper, I.L., Rusin, P., Quintanar, D.R., Haney, C., Josephson, K.L. and Gerba, C.P. (2004). Tracking the concentration of heterotrophic plate count bacteria from the source to the consumer tap. Int. J. Food Microbiol., 92: 289-295.
Percival, S.L. and Williams, D.W. (2014). Legionella. In microbiology of waterborne diseases: Microbiological aspects and risks: Second edition. Elsevier Ltd. Oxford, UK. pp. 155-175.
Pintar, K.D.M. and Slawson, R.M. (2003). Effect of temperature and disinfection strategies on ammonia-oxidizing bacteria in a bench-scale drinking water distribution system. Water Res., 37(8): 1805-1817.
Pinto, A.J., Xi, C. and Raskin, L. (2012). Bacterial community structure in the drinking water microbiome is governed by filtration processes. Environ. Sci. Technol., 46(16): 8851-8859.
Poças, A., Rebola, N., Cordeiro, B., Rodrigues, S., Benoliel, M.J., Vreeburg, J. and Menaia, J. (2013). Methodology for sampling drinking water discolouration loose deposits at low velocities. Water Sci. Technol. Water Supply, 13(4): 1116-1122.
Pons, W., Young, I., Truong, J., Jones-Bitton, A., McEwen, S., Pintar, K. and Papadopoulos, A. (2015). A systematic review of waterborne disease outbreaks associated with small non-community drinking water systems in Canada and the United States. Plos One, 10(10).
Powell, J., J. Clement, M. Brandt, R. Casey, D. Holt, W. Grayman, and M. LeChevallier. (2004). Predictive models for water quality in distribution systems. AwwaRF, Denver, Colorado.
Prest, E.I., Hammes, F., Kötzsch, S., van Loosdrecht, M.C.M. and Vrouwenvelder, J.S. (2013). Monitoring microbiological changes in drinking water systems using a fast and reproducible flow cytometric method. Water Res., 47(19): 7131-7142.
Prest, E.I., Hammes, F., van Loosdrecht, M.C.M. and Vrouwenvelder, J.S. (2016a). Biological stability of drinking water: Controlling factors, methods, and challenges. Front. Microbiol., 7: 45.
Prest, E.I., Hammes, F., Kötzsch, S., Van Loosdrecht, M.C.M. and Vrouwenvelder, J.S. (2016b). A systematic approach for the assessment of bacterial growth-controlling factors linked to biological stability of drinking water in distribution systems. Water Sci. Technol. Water Supply, 16(4): 865-880.
Prest, E.I., Weissbrodt, D.G., Hammes, F., Van Loosdrecht, M.C.M. and Vrouwenvelder, J.S. (2016c). Long-term bacterial dynamics in a full-scale drinking water distribution system. Plos One, 11(10).
Prévost, M., Rompré, A., Coallier, J., Servais, P., Laurent, P., Clément, B. and Lafrance, P. (1998). Suspended bacterial biomass and activity in full-scale drinking water distribution systems: Impact of water treatment. Water Res., 32(5): 1393-1406.
Prince, R.A., Goulter, I. and Ryan, G. (2003). What causes customer complaints about discoloured drinking water? Correlating customer complaints with online monitoring of flow rate and turbidity. Water, 30(2): 62-67.
Proctor, C.R. and Hammes, F. (2015). Drinking water microbiology-from measurement to management. Curr. Opin. Biotechnol., 33: 87-94.
Props, R., Monsieurs, P., Mysara, M., Clement, L. and Boon, N. (2016). Measuring the biodiversity of microbial communities by flow cytometry. Methods in Ecology and Evolution, 7(11): 1376-1385.
Pruden A, Edwards M, Falkinham JO., III (2013). State of the science and research needs for opportunistic pathogens in premise plumbing. Report #4379. Water Research Foundation, Denver, Colorado.
Pryor, M., Springthorpe, S., Riffard, S., Brooks, T., Huo, Y., Davis, G. and Sattar, S.A. (2004). Investigation of opportunistic pathogens in municipal drinking water under different supply and treatment regimes. Water Sci Technol., 50(1): 83-90.
Public Health Agency of Canada. (2021). Notifiable diseases online. Available at: https://diseases.canada.ca/notifiable/
Public Works and Government Services Canada. (2013). MD 15161 - 2013: Control of Legionella in mechanical systems. Standard for building owners, design professionals and maintenance personnel. Available at: https://www.tpsgc-pwgsc.gc.ca/biens-property/documents/legionella-eng.pdf
Quarini, G., Ainslie, E., Herbert, M., Deans, T., Ash, D., Rhys, D., Haskins, N., Norton, G., Andrews, S. and Smith, M. (2010). Investigation and development of an innovative pigging technique for the water-supply industry. Proc. Inst. Mech. Eng. Part E J. Process Mech. Eng., 224(2): 79-89.
Qureshi, N. and Shah, J. (2014). Aging infrastructure and decreasing demand: A dilemma for water utilities. J AWWA, 106 (1): 51-61.
Qin, K., Struewing, I., Domingo, J.S., Lytle, D. and Lu, J. (2017). Opportunistic pathogens and microbial communities and their associations with sediment physical parameters in drinking water storage tank sediments. Pathogens, 6(4): 54.
Ramseier, M.K., von Gunten, U., Freihofer, P. and Hammes, F. (2011). Kinetics of membrane damage to high (HNA) and low (LNA) nucleic acid bacterial clusters in drinking water by ozone, chlorine, chlorine dioxide, monochloramine, ferrate(VI), and permanganate. Water Res., 45(3): 1490-1500.
Rand, J.L., Gagnon, G.A. and Knowles, A. (2014). Establishing minimum free chlorine residual concentration for microbial control in a municipal drinking water distribution system. Water Pract. Technol., 9(4): 491-501.
Rathnayaka, S., Shannon, B., Rajeev, P. and Kodikara, J. (2016). Monitoring of pressure transients in water supply networks. Water Resour. Manage., 30(2): 471-485.
Reasoner, D.J. (2004). Heterotrophic plate count methodology in the United States. Int. J. Food Microbiol., 92(3): 307-315.
Reckhow, D.A., Rees, P.L., Nüsslein, K., Makdissy, G., Devine, G., Conneely, T., Boutin, A. and Bryan, D. (2007). Long-term variability of BDOM and NOM as precursors in watershed sources. Awwa Research Foundation, Denver, Colorado.
Renwick, D.V., Heinrich, A., Weisman, R., Arvanaghi, H. and Rotert, K. (2019). Potential public health impacts of deteriorating distribution system infrastructure. J. Am. Water Works Assoc., 111(2): 42-53.
Revetta, R.P., Gomez-Alvarez, V., Gerke, T.L., Santo Domingo, J.W. and Ashbolt, N.J. (2016). Changes in bacterial composition of biofilm in a metropolitan drinking water distribution system. J. Appl. Microbiol., 121(1): 294-305.
Rhoads, W.J., Garner, E., Ji, P., Zhu, N., Parks, J., Schwake, D.O., Pruden, A. and Edwards, M.A. (2017). Distribution system operational deficiencies coincide with reported legionnaires' disease clusters in Flint, Michigan. Environ. Sci. Technol., 51(20): 11986-11995.
Rittmann, B. E., and Snoeyink, V. L. (1984). Achieving biologically stable drinking water. J. Am. Water Works Assoc., 76: 106-114.
Robertson, P., Abdelhady, H. and Garduño, R.A. (2014). The many forms of a pleomorphic bacterial pathogen-the developmental network of Legionella pneumophila. Front. Microbiol. 5, art. no. 670: 1-20.
Rossman, L.A., Clark, R.M. and Grayman, W.M. (1994). Modeling chlorine residuals in drinking-water distribution systems. J. Environ. Eng., 120(4): 803-820.
Sadiq, R., Kleiner. Y. and Rajani, B. (2009). Proof-of-concept model to predict water quality changes in distribution pipe networks (Q-WARP). Report #2970. AwwaRF, Denver, Colorado.
Safford, H.R. and Bischel, H.N. (2019). Flow cytometry applications in water treatment, distribution, and reuse: A review. Water Res., 151: 110-133.
Saint John Water. (2018). Facts about Saint John's water system. Available at: http://www.saintjohn.ca/site/media/SaintJohn/CSJ_SJW_Backgrounder_SCDWP.pdf
Salter, S.J., Cox, M.J., Turek, E.M., Calus, S.T., Cookson, W.O., Moffatt, M.F., Turner, P., Parkhill, J., Loman, N.J. and Walker, A.W. (2014). Reagent and laboratory contamination can critically impact sequence-based microbiome analyses. BMC Biol., 12(1).
Sarver, E., Zhang, Y. and Edwards, M. (2011). Copper pitting and brass dezincification: chemical and physical effects. Water Research Foundation, Denver, Colorado.
Säve-Söderbergh, M., Bylund, J., Malm, A., Simonsson, M. and Toljander, J. (2017). Gastrointestinal illness linked to incidents in drinking water distribution networks in Sweden. Water Res., 122: 503-511.
Schleich, C., Chan, S., Pullerits, K., Besmer, M.D., Paul, C.J., Rådström, P. and Keucken, A. (2019). Mapping dynamics of bacterial communities in a full-scale drinking water distribution systems using flow cytometry. Water, 11(10): 2137.
Schneider, O.D., Hughes, D.M., Xu, M. and Barfuss, S.L. (2016). Case studies to identify occurrence, accuracy and cause of reverse flow using meter systems. Water Research Foundation, Denver, Colorado.
Scott, D.B., Van Dyke, M.I., Anderson, W.B. and Huck, P.M. (2015). Influence of water quality on nitrifier regrowth in two full-scale drinking water distribution systems. Can. J. Microbiol., 61(12): 965-976.
September, S.M., Els, F.A., Venter, S.N. and Brözel, V.S. (2007). Prevalence of bacterial pathogens in biofilms of drinking water distribution systems. J Water Health, 5(2): 219-227.
Servais, P., Billen, G., Laurent, P., Levi, Y. and Randon, G. (1992). Studies of BDOC and bacterial dynamics in the drinking water distribution system of the northern Parisian suburbs. Revue Des Sciences De l'Eau, 5(Suppl.): 69-89.
Seth, A., Bachmann, R.T., Boxall, J.B., Saul, A.J. and Edyvean, R. (2004). Characterisation of materials causing discolouration in potable water systems. Water Science and Technology, 49(2): 27-32.
Shapiro, H.M. (2003). Practical flow cytometry, fourth ed. Wiley-Liss, New York.
Siebel, E., Wang, Y., Egli, T. and Hammes, F. (2008). Correlations between total cell concentration, total adenosine tri-phosphate concentration and heterotrophic plate counts during microbial monitoring of drinking water. Drinking Water Engineering and Science, 1(1): 1-6.
Singh, I. and Mavinic, D.S. (1991). Significance of building and plumbing specifics on trace metal concentrations in drinking water. Can. J. Civil Eng., 18(6): 893-903.
Skadsen, J., Janke, R., Grayman, W., Samuels, W., Tenbroek, M., Steglitz, B. and Bahl, S. (2008). Distribution system on-line monitoring for detecting contamination and water quality changes. J. Amer. Water Works Assoc. 100(7): 81-94.
Skadsen, J., Reilley, B., Gupta, K., Fiske, P. and Moore, R. (2015). Nitrification control strategies: What is nitrification and how is it managed? Opflow, 41(1): 10-12.
Shurtz, K.M., Jones, S.C., Sowby, R.B., Hill, D.S. and Woodbury, D.K. (2017). Hydraulic modeling finds, fixes chlorine residual gaps. Opflow, 43(6): 28-30.
Smeets, P.W.M.H., Medema, G.J. and Van Dijk, J.C. (2009). The Dutch secret: How to provide safe drinking water without chlorine in the Netherlands. Drink. Water Eng. Sci., 2(1): 1-14.
Speight, V. (2010). Trade-offs between continuous and grab samples for water quality in distribution systems. In: Integrating water systems. Boxall & Maksimovic (eds). Taylor and Francis, London, UK. Pp. 447-451.
Speight, V. (2021). Personal communication. University of Sheffield, Department of Civil and Structural Engineering, Professor of Integrated Water Systems, Sheffield, UK.
Speight, V. and Khanal, N. (2009). Model calibration and current usage in practice. Urban Water J., 6(1): 23-28.
Steger, P. and Pierce, D. (2019). Optimal pump station operation is important, but it isn't easy. Opflow, 45(2): 14-16.
Stoddart, A. (2020). ATP dynamics in drinking water distribution systems. Proceedings of AWWA webinar: Investigating ATP analysis for infrastructure release for service. American Water Works Association, Denver, Colorado.
Strickhouser, A., Falkinham, J. and Edwards, M. (2006). The effects of nutrients and microbial re-growth during storage in domestic water heaters. Proceedings from the AWWA Annual Conference and Exhibit. American Water Works Association, Denver, Colorado.
Sutherns, T. (2019). What's happening underground? Finding leaks & transients in real-time year round. Presented at Ontario Water Works Association Annual Conference and Trade Show, Ottawa, Ontario, May 7.
Sutherns, T. (2020). Gaining visibility into the water distribution system: Predicting leaks + bursts. Presented at American Water Works Association webinar, November 3.
Tafuri, A.N. and Field, R.I. (2010). Aging water infrastructure. Presented at International Workshop on Sustainable Water Environment, Taipei, Taiwan, June 30 - July 1.
Tan, B., Ng, C., Nshimyimana, J.P., Loh, L.L., Gin, K.Y. and Thompson, J.R. (2015). Next-generation sequencing (NGS) for assessment of microbial water quality: Current progress, challenges, and future opportunities. Front. Microbiol., 6(SEP).
Teng, F., Guan, Y.T. and Zhu, W.P. (2008). Effect of biofilm on cast iron pipe corrosion in drinking water distribution system: Corrosion scales characterization and microbial community structure investigation. Corros. Sci., 50(10): 2816-2823.
Tolofari, D.L., Masters S.V., Bartrand, T., Hamilton, K.A., Haas, C.N., Olson, M., Summers, R.S., Rasheduzzaman, M., Young, A., Singh, R. and Gurian, P.L. (2020). Full factorial study of pipe characteristics, stagnation times, and water quality. AWWA Wat. Sci. 2(5), e1204.
Uhl, W. and Schaule, G. (2004). Establishment of HPC(R2A) for regrowth control in non-chlorinated distribution systems. Int. J. Food Microbiol., 92(3): 317-325.
US EPA (2002a). Health risks from microbial growth and biofilms in drinking water distribution systems. Office of Ground Water and Drinking Water, Washington, DC.
US EPA (2002b). Finished water storage facilities. Office of Ground Water and Drinking Water, Washington, DC.
US EPA (2009). Distribution system water quality monitoring: Sensor technology evaluation methodology and results. A guide for sensor manufacturers and water utilities. EPA 600/R-09/076.
US EPA (2013). National primary drinking water standards: Revisions to the total coliform rule. Final rule. Fed. Regist., 78(30): 10269.
US EPA (2016a). Six-year review 3 technical support document for microbial contaminant regulations. Office of Water, Washington, DC. EPA 810-R-16-010. Available at: https://www.epa.gov/sites/production/files/2016-12/documents/810r16010.pdf.
US EPA (2016b). Technologies for Legionella control in premise plumbing systems: Scientific literature review. Office of Ground Water and Drinking Water, Washington, DC. EPA 810-R-16-001
US EPA (2018a). Online water quality monitoring in distribution systems for water quality surveillance and response systems. Office of Ground Water and Drinking Water, Washington, DC. EPA 817-B-18-001.
US EPA (2018b). EPANET: Application for modifying drinking water distribution systems. Available at: www.epa.gov/water-research/epanet
US EPA (2019). Free Chlorine Distribution System Influent Hold Study Protocol. Office of Ground Water and Drinking Water, Washington, DC. EPA 815-B-19-013.
Vaerewijck, M.J.M., Huys, G., Palomino, J.C., Swings, J., and Portaels, F. (2005). Mycobacteria in drinking water distribution systems: ecology and significance for human health. FEMS Microbiol. Rev., 29: 911-934.
van der Kooij, D. (1999). Potential for biofilm development in drinking water distribution systems. J Appl Microbiol., 85: 39S-44S.
van der Kooij, D. (2000). Biological stability: A multidimensional quality aspect of treated water. Water Air Soil Pollut., 123(1-4): 25-34.
van der Kooij, D. (2003). "Managing regrowth in drinking water distribution systems," in Heterotrophic Plate Counts and Drinking Water Safety, eds J. Bartman, J. Cotruvo, M. Exner, C. Fricker, and A. Glasmacher (London: IWA Publishing), 199-232.
van der Kooij, D. and van der Wielen, P.W.J.J. (2014). Microbial growth in drinking-water supplies: Problems, causes, control and research needs. IWA Publishing, London, UK.
van der Kooij, D., Vrouwenvelder, J.S. and Veenendaal, H.R. (2003). Elucidation and control of biofilm formation processes in water treatment and distribution using the unified biofilm approach. pp. 83-90.
van der Kooij, D., Martijn, B., Schaap, P.G., Hoogenboezem, W., Veenendaal, H.R. and van der Wielen, P.W.J.J. (2015). Improved biostability assessment of drinking water with a suite of test methods at a water supply treating eutrophic lake water. Water Res., 87: 347-355.
van der Wielen, P.W.J.J. and van der Kooij, D. (2010). Effect of water composition, distance and season on the adenosine triphosphate concentration in unchlorinated drinking water in the Netherlands. Water Res., 44(17): 4860-4867.
van Lieverloo, J.H.M., Hoogenboezem, W., Veenendaal, G. and van der Kooij, D. (2012). Variability of invertebrate abundance in drinking water distribution systems in the Netherland in relation to biostability and sediment volumes. Water Res., 46(16): 4918-4932.
Van Nevel, S., Koetzsch, S., Weilenmann, H., Boon, N. and Hammes, F. (2013). Routine bacterial analysis with automated flow cytometry. J. Microbiol. Methods, 94(2): 73-76.
Van Nevel, S., Koetzsch, S., Proctor, C.R., Besmer, M.D., Prest, E.I., Vrouwenvelder, J.S., Knezev, A., Boon, N. and Hammes, F. (2017). Flow cytometric bacterial cell counts challenge conventional heterotrophic plate counts for routine microbiological drinking water monitoring. Water Res., 113: 191-206.
Vierheilig, J., Savio, D., Farnleitner, A.H., Reischer, G.H., Ley, R.E., Mach, R.L., Farnleitner, A.H. and Reischer, G.H. (2015). Potential applications of next generation DNA sequencing of 16S rRNA gene amplicons in microbial water quality monitoring. Water Science and Technology, 72(11): 1962-1972.
Viñas, V., Halm, A. and Pettersson, T.J.R. (2019). Overview of microbial risks in water distribution networks and their health consequences: quantification, modelling, trends, and future implications. Can. J. Civ. Eng., 46(3): 149-159.
Vital, M., Stucki, D., Egli, T. and Hammes, F. (2010). Evaluating the growth potential of pathogenic bacteria in water. Appl. Environ. Microbiol., 76(19): 6477-6484.
Vital, M., Dignum, M., Magic-Knezev, A., Ross, P., Rietveld, L. and Hammes, F. (2012). Flow cytometry and adenosine tri-phosphate analysis: Alternative possibilities to evaluate major bacteriological changes in drinking water treatment and distribution systems. Water Res., 46(15): 4665-4676.
Vreeburg, J.H.G., Arsnio, A. and Leijssen, H. (2010). Origin and behavior of particles in drinking water transport networks. Anonymous pp. 453-458.
Wang, Y., Hammes, F., De Roy, K., Verstraete, W. and Boon, N. (2010). Past, present and future applications of flow cytometry in aquatic microbiology. Trends Biotechnol., 28(8): 416-424.
Wang, H., Hu, C., Hu, X., Yang, M. and Qu, J. (2012). Effects of disinfectant and biofilm on the corrosion of cast iron pipes in a reclaimed water distribution system. Water Res., 46(4): 1070-8.
Wang, Z., Choi, O. and Seo, Y. (2013). Relative contribution of biomolecules in bacterial extracellular polymeric substances to disinfection byproduct formation. Environ. Sci. Technol., 47(17): 9764-9773.
Wang, H., Masters, S., Edwards, M.A., Falkinham, J.O. and Pruden, A. (2014a). Effect of disinfectant, water age, and pipe materials on bacterial and eukaryotic community structure in drinking water biofilm. Environ. Sci. Technol., 48(3): 1426-1435.
Wang, H., Proctor, C.R., Edwards, M.A., Pryor, M., Domingo, J.W.S., Ryu, H., Camper, A.K., Olson, A. and Pruden, A. (2014b). Microbial community response to chlorine conversion in a chloraminated drinking water distribution system. Environ. Sci. Technol., 48(18): 10624-10633.
Wang, H., Bédard, E., Prévost, M., Camper, A.K., Hill, V.R. and Pruden, A. (2017). Methodological approaches for monitoring opportunistic pathogens in premise plumbing: A review. Water Res., 117: 68-86.
Warnecke, M. (2006). Cryptosporidium oocyst interactions with drinking water pipe biofilms. Cooperative Research Center for Water Quality and Treatment, Research report 5, Adelaide, AUS.
Whalen, P.A., Whalen, P.J., and Cairns, J.E. (2006). ATP monitoring technology for microbial growth control in potable water systems. In Proceedings of the Conference on Optics and Photonics in Global Homeland Security II, Kissimmee, FL, May 9, Proceedings of SPIE, 6203: 2030N.
Whalen, P.A., Tracey, D.R. and Duguay, J. (2018) Chapter 8: Adenosine triphosphate (ATP) measurement technology. Microbiological Sensors for the Drinking Water Industry, Torben Lund Skovhus and Bo Højris (eds.).
WHO (2003). Heterotrophic plate counts and drinking-water safety: The significance of HPCs for water quality and human health. Published on behalf of the World Health Organization by IWA Pub, London.
WHO (2011a). Guidelines for drinking-water quality. Fourth edition. World Health Organization, Geneva, Switzerland. Available at: https://www.who.int/publications/i/item/9789241549950
WHO (2011b). Water safety in buildings. WHO Press. Geneva, Switzerland. Available at: https://www.who.int/water_sanitation_health/publications/2011/9789241548106/en/
WHO (2014). Water safety in distribution systems. Cunliffe, D. (ed.). World Health Organization, Geneva, Switzerland. Available at: https://www.who.int/water_sanitation_health/publications/water-safety-in-distribution-system/en/
Wilczak, A. (2006). Nitification in drinking water distribution systems. In: Fundamentals and control of nitrification in chloraminated drinking water distribution systems. Manual of water supply practices - M56. Nitification in drinking water distribution systems. 1st edition. American Water Works Association, Denver, Colorado, pp. 21-48.
Wilczak, A., Jacangelo, J.G., Marcinko, J.P., Odell, L.H., Kirmeyer, G.J. and Wolfe, R.L. (1996). Occurrence of nitrification in chloraminated distribution systems. J.Am. Water Works Assoc., 88(7): 74-85.
Wingender, J. and Flemming, H. (2004). Contamination potential of drinking water distribution network biofilms. Water Sci. Technol., 49(11-12): 277-286.
Wingender, J. and Flemming, H. (2011). Biofilms in drinking water and their role as reservoir for pathogens. Int. J. Hyg. Environ. Health, 214(6): 417-423.
Wingender J., Neu T.R. and Flemming H-C. (1999). What are Bacterial Extracellular Polymeric Substances? Chapter 1 in: Microbial extracellular polymeric substances. Wingender J., Neu T.R., Flemming H-C. (eds). Springer, Berlin, Heidelberg.
WRF (2017). Distribution system management: Understanding and controlling biofilms. Fact sheet, updated July 2017. Available at: http://www.waterrf.org/knowledge/distribution-system-management/FactSheets/DistributionSystemMgmt_Biofilms_FactSheet.pdf
Yang, J., LeChevallier, M.W., Teunis, P.F.M. and Xu, M. (2011). Managing risks from virus intrusion into water distribution systems due to pressure transients. J. Water Health, 9(2): 291-305.
Yoder, J., Roberts, V., Craun, G.F., Hill, V., Hicks, L.A., Alexander, N.T. and Radke, V. (2008). Surveillance for Waterborne Disease Outbreaks Associated With Drinking Water and Water Not Intended for Drinking - United States 2005-2006. Morb. Mortal. Wkly. Rep., 57:9:39.
Zacheus, O. M.,Lehtola, M.J.,Korhonen, L.K., and Martikainen, P.J. (2001). Soft deposits, the key site for microbial growth in drinking water distribution networks. Water Res. 35, 1757-1765.
Zahran, S., McElmurry, S.P., Kilgore, P.E., Mushinski, D., Press, J., Love, N.G., Sadler, R.C. and Swanson, M.S. (2018). Assessment of the Legionnaires' disease outbreak in Flint, Michigan. Proc. Natl. Acad. Sci. U. S. A., 115(8): E1730-E1739.
Zhang, Y., Oh, S. and Liu, W.T. (2017). Impact of drinking water treatment and distribution on the microbiome continuum: An ecological disturbance's perspective. Environ. Microbiol., 19(8): 3163-3174.
Appendix A.
List of acronyms
- ASTM
- ASTM International
- ATP
- Adenosine triphosphate
- AWWA
- American Water Works Association
- BOM
- Biodegradable organic matter
- cATP
- Cellular adenosine triphosphate
- DNA
- Deoxyribonucleic acid
- E. coli
- Escherichia coli
- EPS
- Extracellular polymeric substances
- FCM
- Flow cytometry
- HPC
- Hetrotrophic plate count
- NGS
- Next Generation Sequencing
- NRC
- National Research Council
- OPPP
- Opportunistic premise plumbing pathogens
- ORP
- Oxidation-reduction potential
- PCR
- Polymerase chain reaction
- PI
- Propidium iodide
- PVC
- Polyvinyl chloride
- q
- quantitative
- QMRA
- Quantitative microbial risk assessment
- RNA
- Ribonucleic acid
- rRNA
- Ribosomal ribonucleic acid
- US
- United States
- US CDC
- United States Centers for Disease Control and Prevention
- US EPA
- United States Environmental Protection Agency
- WHO
- World Health Organization
Appendix B.
Date | Location, country | Estimated (confirmed) cases | Population served | Causative agent | Possible causes |
---|---|---|---|---|---|
Oct 1980AppB - Footnote b | Grums and Vålberg, Vårmland, Sweden | 2,000 (221) | ~15,000 | Campylobacter jejuni | Cross-connections with factory system; untreated river water was introduced into the distribution system |
Dec 1989-Jan 1990AppB - Footnote b | Cabool, Missouri, USA | 243, 4 deaths |
~2,100 | E. coli O157:H7 | Sewage infiltration during watermains repair and/or water meter replacements |
Nov-Dec 1993AppB - Footnote b | Gideon, Missouri, USA | 650 (31), 7 deaths |
~1,100 | Salmonella typhimirium | Suspected contamination of storage tanks by bird feces; flushing of system drew tank water into service |
1995 | Freuchie, Fife, Scotland | 633 | 1,100 | E. coli O157:H7 | Cross-connection with vegetable processing company; untreated creek water was introduced into the distribution system |
2000 | Strasbourg, France | 53 | 60,000 | Unknown (gastroenteritis symptoms) | Watermain repair in the network |
2000 | Bari, Italy | 344 | 1,000 | Norovirus | Break in pipeline public supply connecting to resort tank |
2000 | Belfast, UK | 117 | Unknown | Cryptosporidium | Seepage of raw sewage from a septic tank into the water distribution system |
2000 | South Wales, UK | 281 | Unknown | Campylobacter | Seepage of surface water contaminated by agricultural waste following heavy rainfall into drinking water reservoir |
2000 | Ohio, USA | 29 | Unknown | E. coli | Possible back-siphonage from an animal barn |
2001 | Darcy le Fort, France | 563 | 1,100 | Cryptosporidium, rotavirus, Campylobacter and E. coli | Sewage contamination occurred in the distribution network upstream to the city |
2001 | Lleida, Spain | 96 | 293 | Norovirus | Contamination of reservoir due to lack of maintenance and structural deficiencies |
2001 | Utrecht, The Netherlands |
37 | 1,866 | Norovirus | Drinking water system connected to grey water system in maintenance work; cross-connection not removed |
2001 | Belfast, UK | 230 | Unknown | Cryptosporidium | Entry of wastewater into the drinking water supply due to a blocked drain |
2002 | Vicenza, Italy | 670 | 3,006 | Unknown (gastrointestinal symptoms) | Broken sewage pipe allowed untreated water from the river to enter the city aqueduct |
2002 | Switzerland | 125 | Unknown | Norovirus | Fecal contamination from sewage leakage |
2004 | Ohio, USA | 1,450 | Unknown | Campylobacter, norovirus and Giardia | Unspecified distribution system deficiency related with untreated groundwater |
2007 | Køge, Denmark | 140 | 5,802 | Campylobacter, E. coli and norovirus |
Technical and human error at sewage treatment plant allowed partially filtered wastewater to enter the distribution system |
2007 | Nokia, Finland | 8,453, 2 deaths |
30,016 | Multiple pathogens Norovirus, Campylobacter and Giardia |
Cross-connection leading to drinking water network contaminated by treated sewage effluent |
2008 | Zurich (Adliswil), Switzerland |
126 | 2,000 | Multiple pathogens Campylobacter and norovirus | Cross-connection leading to input of highly pressurized washwater from sewage plant into the distribution system |
2008 | Northampton, UK | >422 | 250,000 | Cryptosporidium | Dead rabbit found in a storage tank |
2008 | Alamosa, Colorado, USA | 1,300, 1 death |
Unknown | Salmonella | Likely animal contamination of a storage tank |
2009 | Utah, USA | 8 | Unknown | Giardia | Cross-connection between potable and non-potable water sources resulting in backflow |
2010 | Køge, Denmark | 409 | 20,000 | Campylobacter | Contamination of central water supply system by unknown mechanism |
2010 | Öland, Sweden | 200 | Unknown | Norovirus | Untreated water from well in the distribution system |
2010 | Saratoga Springs, Utah, USA | 628 | Unknown | Campylobacter | Cross-connection between potable and non-potable water sources resulting in backflow |
2012 | Kilkis, Greece | 79 | 1,538 | Norovirus | Heavy snowfall and runoff, low temperatures and 15 days without use of school's public water supply increased microbial load |
2012 | Kalundborg, Denmark |
187 | Unknown | Norovirus | Contamination from sewage pipe, due to fall in pressure, during repairs |
2012 | Vuorela, Finland |
800 | 2,931 | Sapovirus and E. coli | Main pipe accidently broken during road construction; flushing after breakage repair proved insufficient and storage reservoir was contaminated |
2013 | Guipuzko, Spain |
238 | 650 | Norovirus and rotavirus | Cross-connection between drinking water supplies and industrial water taken from a river |
|
Footnote
- Footnote 1
-
A combination of interconnected sensors, instruments and other devices, database structure and data analytics that provide real-time status and control of operations.
Page details
- Date modified: