Guidelines for Canadian Drinking Water Quality: Guideline Technical Document – 2,4–Dichlorophenoxyacetic Acid
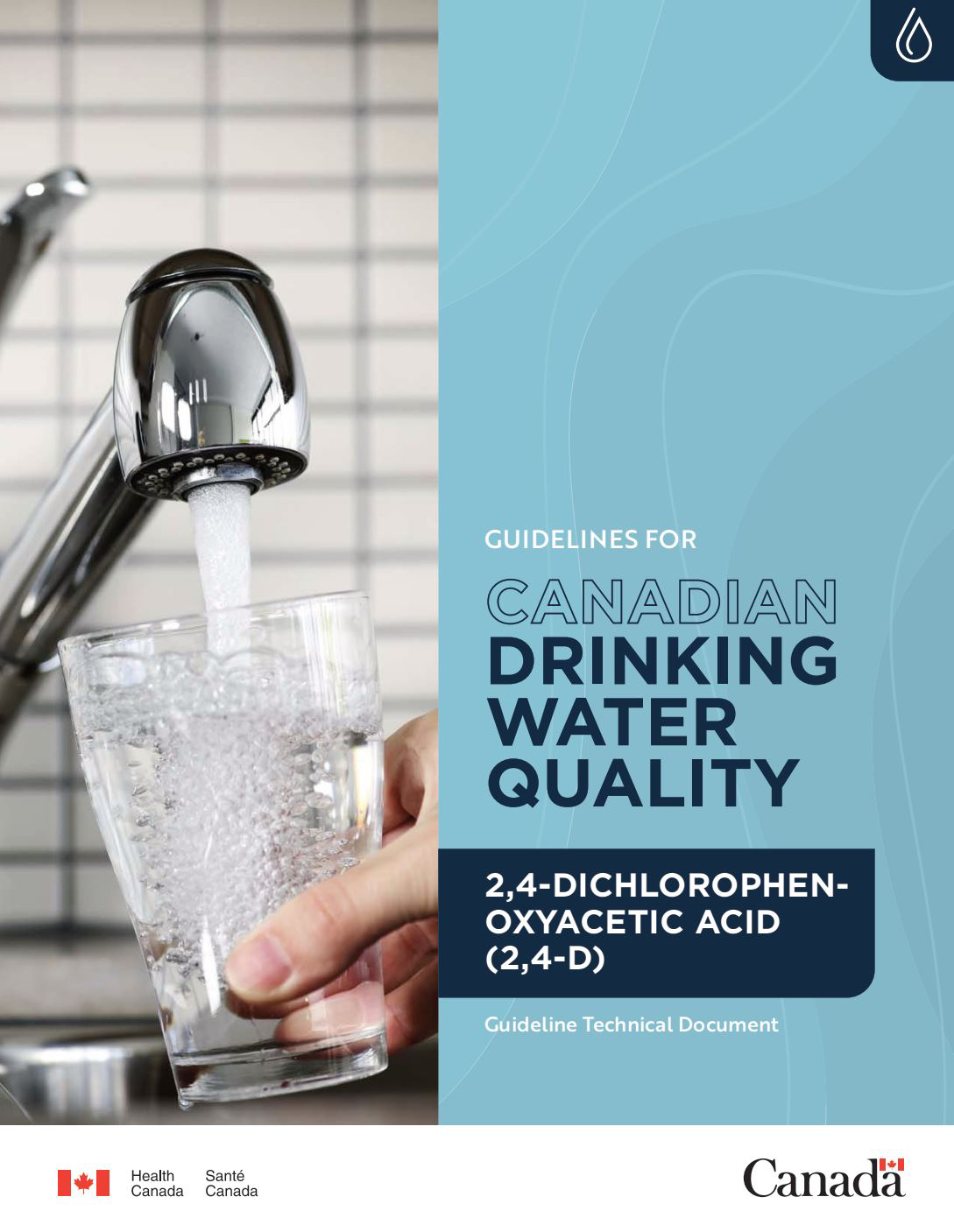
Download the alternative format
(PDF format, 836 KB, 36 pages)
Organization: Health Canada
Type: Guidelines
Date published: 2022-02-04
Related Topics
Guideline
The maximum acceptable concentration (MAC) for 2,4-dichlorophenoxyacetic acid (2,4-D) in drinking water is 0.10 mg/L (100 µg/L).
Executive summary
This guideline technical document was prepared in collaboration with the Federal-Provincial-Territorial Committee on Drinking Water and is based on assessments of 2,4-D completed by Health Canada's Pest Management Regulatory Agency (PMRA) and supporting documents.
Exposure
2,4-D is an herbicide used mainly to control broadleaf weeds. In 2018 (the most recent year for which data are available), it was one of the top 10 active ingredients sold in Canada. It is used on turf, forests, woodlots, terrestrial feed, food crops, and industrial and domestic non-food sites. Various forms of 2,4-D, including the free acid, salts and esters, are used in herbicide formulations and all release the acid as the active ingredient.
Exposure of Canadians to 2,4-D is expected to be low despite the widespread use of 2,4-D. Very low levels of 2,4-D in sources of drinking water have been found in many Canadian provinces. 2,4-D does not tend to accumulate in food, and inhalation exposure is not expected to be significant.
Health effects
Animal studies have consistently found that 2,4-D affects the kidneys of mice and rats. There are no studies regarding kidney effects of 2,4-D in humans. Although some agencies consider 2,4-D to be possibly carcinogenic, international drinking water agencies have all assessed 2,4-D based on its non-cancer effects.
Analytical and treatment
The establishment of a drinking water guideline takes into consideration the ability to both measure the contaminant and remove it from drinking water supplies. 2,4-D can be detected at levels well below the MAC of 0.10 mg/L.
Treatment technologies are available to effectively reduce 2,4-D concentrations in drinking water. Activated carbon adsorption is recognized as the best available technology. Biological filtration processes can also reduce 2,4-D concentrations. However, conventional treatment is not effective for 2,4-D removal. Typical oxidation/disinfection processes used in drinking water treatment also have limited potential to reduce 2,4-D concentrations.
At the residential scale, a number of certified treatment devices are currently available for the removal of 2,4-D. These devices rely mainly on adsorption (activated carbon) and reverse osmosis technologies.
Application of the guidelines
Note: Specific guidance related to the implementation of drinking water guidelines should be obtained from the appropriate drinking water authority in the affected jurisdiction.
The guidelines for 2,4-D are protective against health effects from exposure to 2,4-D in drinking water over a lifetime. Any exceedance of the MAC should be investigated and followed by the appropriate corrective actions if required. For exceedances in source water where there is no treatment in place, additional monitoring to confirm the exceedance should be conducted. If it is confirmed that source water 2,4-D concentrations are above the MAC then an investigation to determine the most appropriate way to reduce exposure to 2,4-D should be conducted. This may include use of an alternate water supply or installation of treatment. Where treatment is already in place and an exceedance occurs, an investigation should be conducted to verify treatment and determine if adjustments are needed to lower the treated water concentration below the MAC.
2,4-D is a chlorophenoxyacetic acid herbicide registered for commercial and domestic use in Canada to control broadleaf weeds. Applications can be made to agricultural crops, forested areas, lawn and turf (including residential uses), and other industrial sites. It is foliar-applied when weeds are actively growing, which, considering the broad use pattern, can be season-long (e.g., spring to fall). In areas of high use, 2,4-D can be introduced into surface water and possibly into groundwater through runoff and infiltration or as the result of spills. 2,4-D is non- to slightly-persistent in water and soil and undergoes rapid biological degradation under aerobic conditions. However, in oxygen-deprived environments such as anaerobic groundwater, the biological degradation of 2,4-D is rather limited.
Table of Contents
- 1.0 Exposure considerations
- 2.0 Health considerations
- 3.0 Derivation of the health-based value
- 4.0 Analytical and treatment considerations
- 5.0 Management strategies
- 6.0 International considerations
- 7.0 Rationale
- 8.0 References
- Appendix A: List of abbreviations
- Appendix B: Canadian water quality data
1.0 Exposure considerations
1.1 Sources and uses
As a selective systemic herbicide and indoleacetic acid (plant hormone) mimic, 2,4-dichlorophenoxyacetic acid or 2,4-D is mainly used to control broadleaf weeds (Charles et al., 1996a; WHO, 2003; US EPA, 2005; Health Canada, 2007). 2,4-D was on Health Canada's Pest Management Regulatory Agency's (PMRA) yearly list of "Top 10 Active Ingredients Sold in Canada" in 2018 (the most recent year for which data are available) with more than 1 000 000 kg of active ingredient (a.i.) (2,4-D) being sold for use on turf, forests, woodlots, terrestrial feed, food crops, and industrial and domestic non-food sites (Health Canada, 2016, 2020). It is foliar-applied when weeds are actively growing, which, considering the broad use pattern, can be season-long (e.g., spring to fall) (Health Canada, 2019).
Because of its low dissociation constant (2.8), 2,4-D will be present in its ionic form under the pH conditions typical of most Canadian soils and water bodies (pH 4.5–8.5). Since its amine salts dissociate in water within a few minutes to the acid anion and a conjugate cation, the environmental behaviour of the amine salts is comparable to that of 2,4-D. 2,4-D and its derivatives are non- to slightly persistent in soil and water. The main transformation products of 2,4-D are carbon dioxide, chlorohydroquinone and 2,4-dichlorophenol as a minor fraction (Health Canada, 2007). 2,4-dichlorophenol is generally biodegradable, highly volatile and non-persistent in aerobic environments (Health Canada, 2007). 2,4-D is short lived in aerobic aquatic environments, with a half-life of less than 2 weeks, depending on water temperature, organic matter, bacterial composition and pH. The laboratory-derived aerobic biotransformation half-life of 2,4-D is 0.22–31 days in soil and 0.25–29 days in water (Health Canada, 2007).
Because of its high water solubility and low organic carbon adsorption coefficient, 2,4-D is expected to readily leach into groundwater if the downward flow of water is rapid. Its soil mobility will increase with increasing pH and decreasing organic content (Johnson et al., 1995; Prado et al., 2001; US EPA, 2005; Health Canada, 2005a, 2007; HSDB, 2015). If the rate of movement of 2,4-D through soil is slow, leaching will be offset by rapid biotransformation in the upper soil horizons and minimal 2,4-D will be found at depth, owing to its relatively short half-life in soil (Health Canada, 2007).
Under anaerobic conditions, biotransformation of 2,4-D is not significant and 2,4-D will persist in soil and aquatic environments. Anaerobic bacterial degradation in groundwater is relatively slow (half-life of 41–1610 days) (Health Canada, 2007).
Bioaccumulation is unlikely based on the low octanol-water partition coefficient (Kow) and rapid degradation of 2,4-D (WHO, 2003; Health Canada, 2007). Spray applications can lead to volatization, especially of the ester forms (Health Canada, 2007).
1.2 Substance identity
2,4-D (CAS Registry No. 94-75-7) is a white crystalline solid with a molecular formula of C8H6Cl2O3 and a molecular weight of 221.0 g/mol (US EPA, 2005; Health Canada, 2016). Based on its physicochemical properties (see Table 1), it is very soluble in water, has a low potential to volatize and rapidly dissociates to its anionic form at environmental pHs (Health Canada, 2005a, 2007). Commercial products contain 2,4-D in a number of different forms: as free acid, as ester (butoxyethyl ester, 2-ethylhexyl ester), as amine (dimethylamine [DMA], isopropylamine [IPA], triisopropanolamine [TIPA]) and as choline salts (Health Canada, 2016). The parent acid (2,4-D) is the herbicidally active portion, while the amine or ester portion allows for greater absorption into the plant (Health Canada, 2005a). The ester and amine forms dissociate quickly (< 3 minutes) to the acid form both in the environment and within biological systems; hence, when 2,4-D is mentioned in this document, it refers to the acid form (Health Canada, 2007). When the other forms are mentioned, their amounts will be expressed as 2,4-D. Diethanolamine and sodium salts of 2,4-D will not be considered in the document as both are not transformation products of 2,4-D and their production and use have been discontinued in Canada (Health Canada, 2006).
2,4-D is often mixed with other herbicides and with additives such as antifoaming agents (Health Canada, 2005a; Kennepohl et al., 2010). In the past, manufacturing processes led to the presence of dioxins (e.g., 2,3,7,8-tetrachlorodibenzo-p-dioxin, 2,3,7,8-tetrachlorodibenzofuran) in 2,4-D preparations; however, with the implementation of new regulatory standards and improved production methods, dioxin levels are similar to or even lower than background levels in the environment (Health Canada, 2005a, 2006; Kennepohl et al., 2010).
Property | 2,4-D | Soluble salts of 2,4-D (DMA, IPA, TIPA) |
InterpretationFootnote a |
---|---|---|---|
CAS# | 94-75-7Footnote b | 2008-39-1, 5742-17-6, 32341-80-3Footnote b | Not applicable |
Molecular formula | C8H6Cl2O3 | C10H13Cl2NO3, C11H15Cl2NO3, C17, H27Cl2NO6 Footnote b | Not applicable |
Molecular weight | 221.0 g/mol Footnote b | 266.13, 280.04, 412.31Footnote b | Not applicable |
n-Octanol/water partition coefficient (log Kow) | 0.04–2.14Footnote a at pH 5 and 25°C | Not available—salts dissociate to acid in water Footnote b | Unlikely to bioaccumulate |
Henry's law constant | 7.26 × 10-6 Pa m3 mol-1Footnote a | Not available—based on DMA salts dissociate rapidly to acidFootnote d | Non-volatile from water or moist surfaces |
Specific gravity | 1.416 at 25°CFootnote b | 1.15–1.23 at 20°C Footnote b | Not applicable |
Water solubility | 24.3 g/LFootnote f at 20°C | 17.4–72.9 g/100 mlFootnote d | Very soluble in water |
Organic carbon adsorption coefficient (Koc) | 20–136Footnote e | 72–136 Footnote c | High potential for leaching |
Dissociation constant (pKa) | 2.8Footnote a | 2.6 (as DMA)Footnote g | Dissociates rapidly to anion at environmental pHs |
Vapour pressure at 25 °C | 1.87 ×10-2 mPaFootnote a | Not available—salts based on DMA salts dissociate to acid in waterFootnote d | Low potential to volatize |
|
1.3 Exposure
As an herbicide, 2,4-D is deliberately applied to food crops. It can potentially be present as a residue in foods and food is likely the main source of non-occupational exposure to 2,4-D for the general Canadian population (Health Canada, 2005a). Levels of 2,4-D in drinking water are often below the detection limit (DL). When detected, levels of 2,4-D in ambient and treated drinking water were generally <1 μg/L (Health Canada, 2005a, 2007). Inhalation exposure is estimated to account for less than 2% of total intake (Kennepohl et al., 2010). Allocating a 20% source contribution to drinking water is deemed appropriate given its minor contribution as a source of exposure (Krishnan and Carrier, 2013).
Water monitoring data from the provinces and territories (municipal and non-municipal supplies), PMRA and Environment and Climate Change Canada (Environment Canada, 2011) were available for 2,4-D and were used to estimate a level of < 1 μg/L for 2,4-D in Canadian drinking (Health Canada, 2007).
Data requested from the provinces and territories show that 2,4-D levels are below the method detection limit or MDL (which ranged from 0.005–1 μg/L) in most samples for groundwater, surface water, raw water, treated water or distribution water where monitoring occurred (Alberta Environment and Sustainable Resource Development, 2013; Manitoba Conservation and Water Stewardship, 2013; New Brunswick Department of Health, 2013; Ministère de l'Environnement et de la Lutte contre les Changements climatiques, 2021; Nova Scotia Environment, 2021; Ontario Ministry of the Environment, 2021; Saskatchewan Water Security Agency, 2021).
In Alberta, 2,4-D was detected at levels of 0.002–1.235 μg/L in 18.4% of treated drinking water samples (75% surface water, 25% groundwater) between 1995 and 2010 (n = 2332, DL = 0.005 μg/L) (Alberta Environment and Sustainable Resource Development, 2013).
In Manitoba, 2,4-D was detected in 13% of surface water in the environment (river, lake, stream) sampled between 2001 and 2012 and the maximum concentration was 8.4 μg/L (n = 1860, DL = 0.005 μg/L) (Manitoba Conservation and Water Stewardship, 2013).
In Nova Scotia, 2,4-D concentrations were measured in raw water (ground and surface water) and treated drinking water between 2013 and 2019. 2,4-D was detected in 2 samples. The maximum concentration was 0.077 µg/L (n= 183; MDL = 0.05–1 µg/L) (Nova Scotia Environment, 2021).
2,4-D was not detected in samples of raw or treated municipal drinking water in New Brunswick (n = 16, MDL = 0.05 µg/L) (New Brunswick Department of Health, 2013).
In Ontario, 2,4-D was detected in 0.16% of the drinking water samples. The maximum concentration of 2,4-D detected in treated surface and groundwater samples taken between 2010 and 2020 was 1 µg/L; the mean was 0.48 µg/L (n = 8 947, DL unspecified) (Ontario Ministry of the Environment, 2021).
Between 2013 and 2018, 2,4-D was detected in 10.8% of the treated drinking water samples in Quebec; the maximum concentration was 0.22 µg/L (n= 2 290 from 175 distribution installations, MDL = 0.03–3.0 µg/L) (Ministère de l'Environnement et de la Lutte contre les Changements climatiques, 2021).
In Saskatchewan, 2,4-D concentrations were measured in surface, ground, treated drinking water, and distribution system samples collected between 2001 and 2020. Only 5 of 1909 samples were above the DL of 0.1–0.5 µg/L; the maximum concentration detected was 12 µg/L and the mean concentration detected was 3.28 µg/L (Saskatchewan Water Security Agency, 2021).
Additional Canadian water monitoring data were also available from the literature. Between April and September of 2007, 19 sites from 16 watersheds across Canada were sampled, including 15 sites downstream from urban centres. 2,4-D was detected in 85% of the 150 samples taken. The mean, median and range of concentrations for all samples of 2,4-D were 172.1 ng/L, 52.7 ng/L and < 0.47–1960 ng/L, respectively (Glozier et al., 2012). Ontario urban streams had significantly higher concentrations of 2,4-D compared with all other areas (p < 0.001). No seasonal differences in stream concentrations of 2,4-D were observed across the country. Samples collected after significant rain events had three-fold higher concentrations of 2,4-D when compared to non-event samples. In Prairie rivers, samples downstream from urban centres had a 1.6-fold increase in 2,4-D when compared with upstream samples (Glozier et al., 2012).
Four rivers from areas in Quebec where corn and soy are intensely cultivated were sampled for 2,4-D from 1993 to 2001 (Giroux, 2002). Annual 2,4-D mean and median concentrations ranged from 0.027 to 0.504 μg/L and from 0.02 to 0.263 μg/L, respectively, while yearly maximum concentrations ranged from 0.2 to 4.1 μg/L (Giroux, 2002).
Between 1998 and 2002, 2,4-D was detected in 12% of Ontario surface water samples (n = 262) taken from the Don River and Humber River watersheds. The mean concentration was 0.13 μg/L (MDL = 0.1 μg/L) and the maximum concentration was 3.2 μg/L (Struger and Fletcher, 2007). 2,4-D was present in drinking water above the DL (0.05–0.1 µg/L) in only 1% (1/122) of the kitchen taps of homes sampled as part of the Ontario Farm Family Health Study (Arbuckle et al., 2006). Under Environment Canada's Great Lakes Surveillance Program, surface water samples were taken from all Great Lakes except Michigan between 1994 and 2000. 2,4-D concentration ranges were 2.3–14.5 ng/L, <0.40–84.4 ng/L, <0.29–1.4 ng/L, and <0.40 to 2.5 ng/L for lakes Ontario, Erie, Huron, and Superior, respectively (Struger et al., 2004).
Reservoirs and associated treated drinking water from Manitoba, Saskatchewan, and Alberta (total of 15 locations) were sampled for 2,4-D from 2003 to 2005 (Donald et al., 2007). All samples tested (n = 206) were above the limit of detection (LOD) of 0.47 ng/L. Drinking water had a mean annual concentration of 75 ng/L and a maximum concentration of 589 ng/L, while reservoir water had a mean concentration of 123 ng/L and a maximum concentration of 1850 ng/L (Donald et al., 2007).
No information was found regarding 2,4-D levels in Canadian foods. According to the World Health Organization (WHO) (WHO, 2003) "Available evidence indicates that residues of 2,4-D rarely exceed a few tens of μg/kg in food"; thus 2,4-D is unlikely to accumulate in food. Health Canada (2018a) has set maximum residue limits for a variety of food products (including fruits, vegetables, and animal tissues/organs) of 0.01–5 ppm of 2,4-D. Estimates of the dietary exposure to 2,4-D have been calculated using average consumption of different foods, average residue values on those foods over a 70-year lifetime and the different eating habits of the population at various stages of life. The general population, infants/children, and youth (ages 7–12 years) were estimated to have intakes of 0.12, 0.27, and 0.16 µg/kg body weight (bw) per day of 2,4-D from food, respectively (Health Canada, 2007).
In general, exposure to 2,4-D from air is considered to be low. 2,4-D is most often detected in the air immediately following application (Tuduri et al., 2006). Interim results from a 3-year national air surveillance program by the Canadian Atmospheric Network for Currently Used Pesticides (CANCUP) showed that atmospheric concentrations of 2,4-D varied within years and time periods, and by regional characteristics (Yao et al., 2008). Atmospheric samples were collected at 8 sites (6 agricultural, 1 wetland, 1 urban) across Canada in 2004 and 2005. Average concentrations were 10.0–730 pg/m3 in 2004 and 59.4–193 pg/m3 in 2005 (MDL = 1.4 pg/m3). Levels of 0–5 ng/m3 were measured in air following turf spraying of 2,4-D in 3 suburbs of Québec City between 2001 and 2002 (Giroux and Therrien, 2005). Levels recorded in Saskatchewan between 1989 and 2002 were 190–2,730 pg/m3; peak levels were recorded in June, followed by progressively decreasing levels (Tuduri et al., 2006). In Alberta, the Air Research Users Group of Alberta Environment reported that most concentrations were below 0.1 ng/m3 and the maximum concentration was 0.36 ng/m3 (n = 4 locations; MDL = 0.05 mg/m3) (Kumar, 2001).
2,4-D was not detected at any sampling site of the CANCUP surveillance study in 2005 (n = 8 locations, 4 samples per location, MDL = 0.2 ng/g) (Yao et al., 2008).
Most Canadians have very low urine levels of 2,4-D, as measured in Cycle 1 (2007–2009) and Cycle 2 (2009–2011) of the Canadian Health Measures Survey (Health Canada, 2013). In the Canadian Health Measures Survey group geometric means of urinary 2,4-D were not calculated if more than 40% of the samples were below the DL of 0.2 µg/L. When the data were stratified by sex and age, geometric means could only be calculated for Cycle 2 males in the age groups of 6–11, 20–39 and 40–59 years, as well as the male total age group (3–79 years); the range was 0.24–0.29 µg/L (95% confidence interval or CI: 0.20–0.39 µg/L).
The Ontario Farm Family Health Study and the Pesticide Exposure Assessment Pilot Study reported mean urinary 2,4-D concentrations of 1.0–40.8 µg/L in farm applicators (n = 126; 20% below the LOD), of 0.7–2.0 µg/L in farmers' wives (n = 125; 84% below the LOD), and of 0.7–2.9 µg/L in their children (n = 92; 70% below the LOD) (Arbuckle et al., 1999b, 2004, 2005, 2006; Arbuckle and Ritter, 2005).
2.0 Health considerations
All pesticides, including 2,4-D, are regulated by PMRA. PMRA conducts extensive evaluations and cyclical reviews of pesticides, including unpublished and proprietary information, as well as foreign reviews by other regulatory agencies such as the United States Environmental Protection Agency (US EPA). As such, this health assessment is primarily based on PMRA's evaluations and supporting documentation (Health Canada, 2005a, 2005b, 2006, 2007, 2018b, 2018c). Additionally, reviews and relevant literature available since PMRA's evaluations were completed were also considered.
2.1 Kinetics
The pharmacokinetics of 2,4-D is fairly consistent across species, with the exception of dogs which have a longer plasma half-life due to their slower renal transport clearance mechanism and to their inability to excrete organic acids (van Ravenzwaay et al., 2003; Timchalk, 2004). Because of their substantially higher body burden at comparable doses, canine data may not be relevant to human health risk assessment (Timchalk, 2004).
The pharmacokinetics of 2,4-D following dermal absorption is different from that following oral ingestion (Kennepohl et al., 2010). Given that dermal absorption is not a significant route of exposure via drinking water, pharmacokinetic studies using dermal exposure are not further considered. As the salts and ester compounds of 2,4-D are rapidly hydrolyzed to the acid in water after absorption, only the pharmacokinetics of the acid form of 2,4-D will be discussed (Frantz and Kropscott, 1993).
Absorption: 2,4-D is readily absorbed from the gastrointestinal tract (92%–99%) following oral ingestion in rats and humans, with the rate of absorption decreasing with increasing dose (Sauerhoff et al., 1977; Gorzinski et al., 1987; Timchalk et al., 1990; Kennepohl et al., 2010). Absorption rate was unaffected by sex in a study using Sprague-Dawley rats orally dosed with 5 or 200 mg/kg of 2,4-D (Griffin et al., 1997).
In humans, peak plasma concentrations of 25–40 µg/mL were attained between 7 hours and 24 hours after ingestion of capsules containing 5 mg/kg bw of 2,4-D by 6 healthy male volunteers (Kohli et al., 1974). In Sprague-Dawley rats given 5 mg/kg bw of 2,4-D orally, peak blood concentrations of 7.5 µg/L for males and 16.2 µg/L for females were reached in 26.8 minutes and 42.9 minutes, respectively (Griffin et al., 1997).
Distribution: Being very hydrophilic, 2,4-D rapidly distributes throughout the body but does not appear to accumulate in any tissue with repeated dosing (Erne, 1966; Munro et al., 1992; OEHHA 2009; Kennepohl et al., 2010). 2,4-D is highly bound (93%–97%) to plasma proteins over a broad range of concentrations (Timchalk, 2004). Distribution was similar in several species (mice, hamsters, rats, pigs, calves, chickens) that were orally administered 2,4-D, with the highest acculmulation reported in the liver, kidney, lung and spleen. The values sometimes exceeded that found in plasma (Erne, 1966; Griffin et al., 1997). Low levels were also seen in the gonads, fat and brain (Griffin et al., 1997). Penetration of 2,4-D into adipose tissue and into the central nervous system was limited and likely influenced by 2,4-D existing predominantly in the ionized form at physiological pH making it unable to readily cross lipid membranes (Erne, 1966; Munro et al., 1992). Over time, the concentration of 2,4-D in the kidneys eventually exceeded the levels detected in the blood/plasma, reflecting the importance of the kidneys as the primary route of 2,4-D elimination (Timchaulk, 2004). In rats given single oral doses, 2,4-D levels in all tissues peaked at 6 hours and then dropped rapidly over 24 hours (Munro et al., 1992).
In animal studies using radiolabelled single doses, 2,4-D was reported to cross the placenta and was detected in the fetuses of rats and mice. However, elimination from the fetuses was rapid—within 24 hours (Munro et al., 1992).
Metabolism: 2,4-D is not extensively metabolized in rats and humans regardless of the exposure dose, dosing duration or route administered (Kohli et al., 1974; Munro et al., 1992). Only the parent compound was found in the urine and feces of rats after oral administration of 5–200 mg/kg bw of 2,4-D (Frantz and Kropscott, 1993; Griffin et al., 1997; van Ravenzwaay et al., 2003). In hamsters and mice, 2,4-D is the main compound found in urine, although conjugates (i.e., glycine and taurine in mice; glycine, taurine and glucuronide in hamsters) are also present at various levels (Griffin et al., 1997; van Ravenzwaay et al., 2003).
Elimination: Renal excretion is reported as the main route of 2,4-D elimination in humans, rats, hamsters and mice, while feces and expiration represent minor routes (Timchalk et al., 1990; Kennepohl et al., 2010). 2,4-D is secreted by the renal proximal tubules in rats and humans, using an active transport system that is saturable (Hasegawa et al., 2003; Nozaki et al., 2007; Kennepohl et al., 2010). In rats, saturation occurs at 50–60 mg/kg bw (Gorzinski et al., 1987; Hasegawa et al., 2003; Nozaki et al., 2007; Kennepohl et al., 2010). The rate of urinary excretion is inversely proportional to the dose administered (Kennepohl et al., 2010). In humans, the parent compound was detected in the urine as soon as 2 hours following a single oral administration of 5 mg 2,4-D/kg bw, and 75% was excreted unchanged within 96 hours (Kohli et al., 1974). The average half-life in humans following ingestion ranges from 18 to 40 hours, although a high value of 220 hours has been reported (Friesen et al., 1990). The variability in half-life is likely related to differences in urine pH and its effect on renal clearance (Friesen et al., 1990). Rats given single oral doses of 10, 50 or 150 mg/kg bw of 2,4-D showed a biphasic clearance with mean excretion half-lives of 0.9 hours for the alpha phase and of 18 hours for the beta phase (Smith et al., 1990). Excretion reached saturation at 50 mg/kg bw (Smith et al., 1990; van Ravenzwaay et al., 2003). Female rats exposed to 200 mg/kg bw had a longer elimination half-life (139.4 hours) than males (34.6 hours) (Griffin et al., 1997).
2.2 Health effects
With very few exceptions (most notably diethanolamine), the effects and relative toxicities of the salt and ester forms of 2,4-D are quite similar to those of the acid form (Health Canada, 2005a; US EPA, 2005).
2.3 Effects in humans
Although some epidemiological studies have shown associations between exposure to 2,4-D and the risk of cancer, birth defects or Parkinson's disease, study shortcomings (e.g., improper exposure measurements, other confounding factors such as co-exposure to other pesticides or contaminants, small sample sizes) hinder any definitive conclusions.
Information on acute toxicity is limited to cases of accidental and intentional ingestion of 2,4-D. These cases mostly involved ingesting mixtures containing 2,4-D in combination with other herbicides (e.g., dicamba, methylchlorophenoxyacetic acid) and/or with solvents and emulsifiers used in various formulations, making it difficult to differentiate the toxicity of 2,4-D from that of the other chemicals. Additionally, patients often vomit following ingestion. Both the ingestion of mixtures and the presence of vomiting could account for the wide range of oral median lethal dose (LD50) values for humans (300–1000 mg/kg bw) reported in the literature (Nielsen et al., 1965; Kancir et al., 1988; Friesen et al., 1990; Durakovic et al., 1992; Bradberry et al., 2000; Brahmi et al., 2003). Symptoms following oral ingestion of mixtures containing 2,4-D have been reported in a number of organs and organ systems, including the kidney, the central nervous system, the gastrointestinal tract and the cardiovascular system; death resulted from severe multiple organ failure or cardiac arrest in cases of fatal poisonings (O'Reilly, 1984; Kancir et al., 1988; Flanagan et al., 1990; Friesen et al., 1990; Durakovic et al., 1992; Keller et al., 1994; Bradberry et al., 2000; Brahmi et al., 2003). No signs of toxicity were observed in five healthy male volunteers given a single 5 mg/kg bw dose of analytical grade 2,4-D (Sauerhoff, 1977).
Cancer: Although some epidemiological studies have observed associations between exposure to 2,4-D and non-Hodgkin's lymphoma, soft-tissue sarcoma, and prostate and gastric cancers in industrial and agriculture workers (Miligi et al., 2003, 2006; Mills et al., 2005; Mills and Yang, 2007), other studies have failed to support such associations (Wiklund et al., 1987; De Roos et al., 2003; Eriksson et al., 2008; Goodman et al., 2015, 2017). The absence of direct measures of individual exposure, the small cohort sizes and the presence of other contaminants, such as other pesticides, make it difficult to draw definitive conclusions. Moreover, the vast majority of the recent follow-up and meta-analysis studies have found no relationship between 2,4-D exposure and gastric, prostate or soft-tissue cancers (Bloemen et al., 1993; Kogevinas et al., 1997; Burns et al., 2011; Pahwa et al., 2011; Burns and Swaen, 2012; Goodman et al., 2017).
Finnish workers spraying 2,4-D exclusively did not have an increased number of chromosomal aberrations in peripheral lymphocytes. The length of exposure ranged from 9 to 28 days. Urinary levels of 2,4-D taken at the end of exposure ranged from 0.02 to 1.56 mg/L and showed large variability among individuals. The use of personal protective equipment was not specified (Mustonen et al., 1986).
Non-Cancer: Although a relationship between exposure to 2,4-D and Parkinson's disease has been suggested in older studies, these studies often looked at overall pesticide exposure rather than at a specific pesticide (Semchuk et al., 1992). With the exception of Tanner et al. (2009), most recent studies have not found an association between Parkinson's disease and exposure to 2,4-D (Kamel et al., 2007; Dhillon et al., 2008; Hancock et al., 2008; Rugbjerg et al., 2011; Burns and Swaen, 2012). In Tanner et al. (2009), an occupational case-control study, a slight increase in the odds of Parkinson's disease and the use of 2,4-D in men from 8 North American clinics was observed (OR = 2.59, 95% CI = 1.03–6.48); however, the study had a number of weaknesses, including a risk of recall bias, poorly characterized exposure, co-exposure of some subjects to other pesticides and a lower limit of the confidence interval greater than 1.0.
Developmental and reproductive toxicity: The interpretation of epidemiological results for potential developmental and reproductive effects was often confounded by factors such as the use of pesticide formulations, the general grouping of 2,4-D with other pesticide classes or with all phenoxy herbicides, the use of indirect measures of exposure, unmeasured confounding factors and biases, and, in older studies, pesticide contamination with dioxins (Health Canada, 2007).
In the Ontario Farm Family Health Study, a retrospective questionnaire-based study carried out in 2000 in farm couples, pre- and post-conception exposure to phenoxy herbicides did not increase the odds of spontaneous abortion in the first trimester (Arbuckle et al., 1999a). In a study by Garry et al. (1996), the odds of central nervous system, circulatory/respiratory, urogenital and muscular anomalies in newborns (n = 4935) of pesticide applicators (n = 34 772) in Minnesota were significantly associated with combined chlorophenoxy herbicide/fungicide exposure (OR = 1.86, 95% CI = 1.69–2.05); no individual exposure data were available for 2,4-D.
In a poorly described study, sperm analysis (volume, count, mobility, morphology) of 32 male farm sprayers exposed to 2,4-D (mean urinary concentration of 9.02 mg/L of 2,4-D) revealed significant decreases in sperm motility and in the number of live sperm, as well as a significant increase in abnormal sperm morphology when compared with unexposed controls (n = 25; no detectable 2,4-D in urine). However, results were inconsistent across exposure periods and information on timing of urine and semen collection was missing (Lerda and Rizzi, 1991). In an in vitro study using spermatozoa from healthy volunteers, doses of ≥ 10 µM of 2,4-D resulted in dose-dependent decreases in total motility, progressive motility and capacitation in the presence of progesterone, while doses of ≥ 1 µM decreased the ability of sperm to penetrate a viscous medium (Tan et al., 2016). Doses of up to 200 µM had no effect on sperm viability, capacitation without progesterone, or acrosome reactions (Tan et al., 2016). The authors hypothesized that 2,4-D could alter intracellular calcium concentrations and induce oxidative stress (Tan et al., 2016).
2.4 Effects in animals
Adverse effects of 2,4-D observed in subchronic and chronic animal studies included kidney, liver, and retinal toxicity, changes in body and organ weights (thyroid, kidney, adrenals), and alterations in blood chemistry and thyroid hormone levels (Serota et al., 1983a, 1983b; Gorzinski et al., 1987; Schultze, 1991a, 1991b; Jeffries et al., 1995; Charles et al., 1996b; Mattsson et al., 1997; Marty et al., 2013; Neal et al., 2017). There was no evidence that 2,4-D compounds caused carcinogenicity in animals. 2,4-D generally did not induce reproductive or developmental effects in rodents except at maternally toxic doses (Jeffries et al., 1995; Charles et al., 1996a; Kennephol et al., 2010; Marty et al., 2013; Pochettino et al., 2016).
Orally administered 2,4-D caused moderate acute toxicity in animals (Carreon et al., 1983; Gorzinski et al., 1987). In a study by Mattsson et al. (1997) single, gavage doses of 15, 75 or 250 mg/kg of 2,4-D were given to groups of 10 rats per sex. A slight to minimal alteration in gait was observed on day 1 in 2 rats at 75 mg/kg. A dose of 250 mg/kg bw caused slight, transient changes in gait and in coordination which resolved by day 8. A no-observed-adverse-effect level (NOAEL) of 15 mg/kg was determined by the authors. The oral LD50 values for 2,4-D were 607 and 726 mg/kg bw for male and female F344 rats, respectively (Gorzinski et al., 1987). Different forms of 2,4-D had similar or lower oral toxicity when considered as the active ingredient (a.i.), with rat values of 536 and 424 mg/kg bw a.i. for males and females, respectively for isobutyl ester and 619 and 490 mg/kg bw a.i. for males and females, respectively, for DMA (Gorzinski et al., 1987). The IPA salts were less acutely toxic, with oral LD50 values of 1,646 and 2,322 mg/kg bw for female and male rats, respectively (Carreon et al., 1983).
Kidney effects: Renal effects consisting of slightly altered clinical chemistry and kidney weights were seen in a 2-year chronic toxicity/oncogenicity study in which F344 rats (60/sex/dose) were fed diets containing 0, 5, 75 or 150 mg/kg bw per day of technical grade 2,4-D (purity 96.4%) with an interim sacrifice of 10 rats/sex/dose at 12 months (Jeffries et al., 1995; Charles et al., 1996a). Animal survival was unaffected by treatment. At 2 years, kidney weights were unaffected in males, whereas females had a slight, statistically significant decrease at 150 mg/kg bw per day and a statistically significant increase in kidney to body weight ratio at ≥ 75 mg/kg bw per day. Decreased food consumption was seen in females at ≥75 mg/kg bw per day and in males at 150 mg/kg bw per day; it was accompanied by decreased weight gain. At ≥ 75 mg/kg bw per day, blood urea nitrogen (BUN) was decreased in males only, while creatinine was increased in females only. At interim sacrifice, both males and females dosed with ≥ 75 mg/kg bw per day had degeneration of the proximal convoluted tubules, but no effect on kidney histopathology was noted in rats sacrificed at 2 years (Jeffries et al., 1995; Charles et al., 1996a).
A similar 2-year dietary study using F344 rats (60/sex/dose) dosed with 0, 1, 5, 15 or 45 mg/kg bw per day of 2,4-D showed no effect on clinical chemistry, gross pathology or survival (Serota, 1986). Relative body weight gain in high-dose females was significantly reduced at 12 and 24 months, although food consumption was decreased at 12 months only. Absolute and relative kidney weights were increased in both males (≥ 15 mg/kg bw per day) and females (all doses) at 2 years. Renal pelvic mineralization was seen in females starting at 15 mg/kg bw per day. Changes in kidney histopathology (increased incidence of brown tubular pigment and severity of fine cytoplasmic vacuolization in the renal cortex) were seen in both sexes starting at 5 mg/kg bw per day, although a review by an independent pathology working Group at Research Triangle Park involving re-sectioning and reading kidney slides from the study found no difference in tubular cell pigmentation between the dosed and control groups (Health Canada, 2005b). The pathology working group also concluded that the nature of the pigment in all study animals was morphologically similar to that known to occur spontaneously in F344 rats of this age. The incidence and severity of pelvic mineralization was considered to be treatment-related in males at the 45 mg/kg bw and in females at 15 and 45 mg/kg bw (Health Canada, 2005b).
In a 2-year dietary study, B6C3F1 female mice were given 0, 5, 150 or 300 mg/kg bw per day of 2,4-D while males were given 0, 5, 62.5 and 125 mg/kg bw per day (Jeffries et al., 1995; Charles et al., 1996a). Dose-related increases in absolute and relative kidney weights were noted in both sexes at the two highest doses and were associated with minimal alterations in the descending proximal tubules of the kidneys. Vacuolization of the proximal tubules was also observed at these doses.
The kidney was the target organ in an extended one-generation dietary study (preceding the publication of OECD guideline 443) in which Crl:CD rats (27/sex/dose; parental generation or P1) were fed 2,4-D at 0, 7, 21 or 40 mg/kg bw per day until the end of lactation for females or 0, 6, 17 or 45 mg/kg bw per day until 7 weeks post mating for males (Marty et al., 2013). Diets were continued in the offspring (F1) up to postnatal day (PND) 139, with interim sacrifices at PNDs 60, 70 and 90. Statistically significant increases in absolute and relative kidney weights were seen in high-dose P1 males and in high-dose F1 females at PND 139. Renal lesions were seen in high-dose P1 males, in mid-dose F1 males and in high-dose F1 animals (both sexes); they were characterized by very slight to slight degeneration of the proximal convoluted tubules. Lesions were more severe in males than females.
Liver effects: In a 2-year chronic toxicity/oncogenicity study, F344 rats (60/sex/dose) were fed diets containing 0, 5, 75 or 150 mg/kg bw per day of technical grade 2,4-D (purity 96.4%); there was an interim sacrifice of 10 rats/sex/dose at 12 months (Jeffries et al., 1995; Charles et al., 1996a). Animal survival was unaffected by treatment. In both sexes, liver weights were significantly decreased at the two highest doses while alkaline phosphatase (ALP) was significantly increased. Alanine transaminase (ALT)Footnote 1 and aspartate transaminase (AST)Footnote 2 were significantly increased starting at ≥75 mg/kg bw per day in males only. Histopathological examination showed minimal panlobular discolouration in the liver, although the authors did not consider the finding to be toxicologically significant.
Liver effects (changes in clinical chemistry and histology) have been reported in F344 rats fed 150 mg/kg bw per day and in B6C3F1 mice fed 300 mg/kg bw per day of 2,4-D for 13 weeks (Serota et al., 1983a, 1983b; Gorzinski et al., 1987; Schultze, 1991a, 1991b). Decreases in ALT, AST, ALP activities and BUN were observed in rats of both sexes exposed to 15 and 45 mg/kg bw per day for 13 weeks (Serota et al., 1983a). In another 13-week study, rats receiving the top dose of technical grade 2,4-D (150 mg/kg bw per day) had a slight statistically significant increase in ALT in both sexes, while ALP and relative liver weights were slightly increased in females only (Gorzinski et al., 1987). Both sexes showed minor, nonspecific hepatocellular changes at the two highest doses (100 and 150 mg/kg bw per day) (Gorzinski et al., 1987). Similarly, ALT and AST levels increased in male and female rats exposed to 2,4-D at 100 and 300 mg/kg bw per day (doses ranged from 1–300 mg/kg bw per day for 13 weeks) and were accompanied by increased liver weight, liver lesions and centrilobular hepatocellular hypertrophy (Schultze, 1991b). Mice exposed to 1–300 mg/kg bw per day (both sexes) for 13 weeks also had histopathological lesions of the liver (characterized as nuclear hyperchromatism), and decreased glycogen in periportal hepatocytes but only at the highest dose given (Schultze, 1991a).
Endocrine effects: Increased thyroid weights, non-significant histopatholgical changes (parfollicular cell nodular hyperplasia), and decreased thyroxin (T4) levels were seen starting at 75 mg/kg bw per day in a 2-year chronic toxicity/oncogenicity study in F344 rats fed diets containing 0, 5, 75 or 150 mg/kg bw per day of 2,4-D (Jeffries et al., 1995; Charles et al., 1996a). T4 levels were also significantly decreased in the two highest dose groups of F344 rats (females only) fed diets containing 0, 15, 60 and 150 mg/kg bw per day of 2,4-D for 13-weeks (Gorzinski et al., 1987). In another 13-week F344 rat feeding study, absolute and relative thyroid weights were increased in males at all doses (1, 5, 15, or 45 mg/kg bw per day of 2,4-D) and in females at the three highest doses, while T4 levels were increased in males at 5 and 15 mg/kg bw per day (Serota et al., 1983a). In a comprehensive one-generation reproductive study in CD rats examining androgen, estrogen, and thyroid endpoints, endocrine-related effects were limited to slight thyroid hormone changes in pregnant dams only and were considered adaptive by the authors (Marty et al., 2013). Increased adrenal gland weights were observed in female mice at the 5 mg/kg bw per day and in F344 rats of both sexes at 100 and 300 mg/kg bw per day; changes in rats were correlated with cellular hypertrophy of the zona glomerulosa (Serota et al., 1983b; Schultze, 1991a).
Based on a recent comprehensive review of in vitro and in vivo studies, 2,4-D has a low potential to interact with the endocrine system (Neal et al., 2017). The review used a weight-of-evidence approach. It had a detailed protocol for literature search and for inclusion and evaluation of the quality of both regulatory and published mammalian toxicological and epidemiological studies. It also considered the coherence and consistency of findings and potential modes of action and it assessed the database for deficiencies. The review found no interactions between 2,4-D and endocrine pathways (estrogen, androgen, steroidogenesis or thyroid) (Neal et al., 2017). Results from five in vitro assays used by the US EPA's Endocrine Disruptor Screening Program were also negative for effects on androgen, estrogen, and steroidogenesis pathways of the endocrine system (Coady et al., 2014).
Eye effects: In subchronic and chronic feeding studies in F344 rats, 2,4-D and its esters caused histopathological lesions of the eyes (cataracts and retinal degeneration) at the highest doses tested (300 mg/kg bw per day in 13-week studies; 150 mg/kg bw per day in 1- and 2-year studies) (Schultze et al., 1991a; Szabo and Rachunek, 1991; Charles et al., 1996b; Mattsson et al., 1997).
Neurotoxicity: In addition to the retinal degeneration mentioned above, the 2-year neurotoxicity study in rats by Mattson et al. (1997) found treatment-related alterations in grip strength relative to body weight at 150 mg/kg bw per day of 2,4-D (65 rats/dose; 0, 5, 75, and 150 mg./kg bw per day). There were no treatment-related effects on functional observational battery, landing foot splay or motor activity. The authors considered 75 mg/kg bw per day to be the NOAEL for chronic neurotoxicity.
Reproductive/developmental toxicity: In animal studies, reproductive effects were only observed at doses that exceeded renal clearance, while fetotoxic effects occurred only at maternally toxic doses (Rodwell, 1985; Charles et al., 2001; Marouani et al., 2017).
No evidence of reproductive toxicity or developmental neurotoxicity was found in an Extended One-Generation Reproductive Toxicity Study (pre-OECD guideline 443) in which CD rats were fed 2,4-D (6–45 mg/kg bw per day) during critical windows of development (pre-mating, mating, gestation and lactation) (Marty et al., 2013).
Litter size and resorption rates were unaffected in a series of studies in pregnant rats (8–150 mg/kg bw per day as 2,4-D from gestation day GD 6–15) and pregnant rabbits (10–90 mg/kg bw per day as 2,4-D from GD 6–18) gavaged with 2,4-D, its salts and its esters (Charles et al., 2001). Significant fetal effects (decreased fetal body weights and increased fetal variations) were seen in rats given maternally toxic doses (≥ 90 mg/kg bw per day as 2,4-D) but not in rabbits. PMRA (Health Canada, 2007) considered both the maternal and the developmental NOAELs for rats to be 25 mg/kg bw per day as 2,4-D.
No effects on body weight gain, number of pups born, pup postnatal mortality, or growth hormone levels were observed in pregnant rats given 70 mg/kg bw per day of 2,4-D in the diet from GD 16 until PND 23 or in their weanling pups fed the same diet until PND 45, 60, or 90 (Pochettino et al., 2016).
No sign of maternal toxicity was observed in pregnant CD-1 mice given a 2,4-D-amine derivative at 8.5, 37 or 370 mg/kg bw per day as 2,4-D in drinking water on GD 6–16 although female pups had decreased body weight and minor reductions in the kidney weights at 37 and 370 mg/kg bw per day (Lee et al., 2001).
Reproductive toxicity was seen at high doses in a multigeneration reproductive toxicity study in F344 rats (30/sex/dose) fed diets containing 0, 5, 20, or 80 mg/kg bw per day of 2,4-D for 105 days before mating (F0 generation), and continuing throughout mating, gestation and lactation (F0 and F1 generations) (Rodwell, 1985). The F0 generation produced 2 litters, F1A and F1B. The F1B litter became the F1 generation and produced F2A and F2B litters. The high dose of 80 mg/kg bw per day was discontinued during the F1 generation as it caused excessive parental toxicity, including significantly decreased mean body weights in adults and in adult offspring. Histopathological examination revealed increased focal nuclear density in the medullary renal tubules in animals of the F0 generation at 20 and 80 mg/kg bw per day and in the F1 adults at 20 mg/kg bw per day when compared with controls. Pup body weights were significantly lower in the F1B generation at 20 and 80 mg/kg bw per day while the weights of F1A pups were significantly decreased at 80 mg/kg bw per day. Viability of F1A and F1B pups was affected only at 80 mg/kg bw per day. Live litter sizes were reduced in the F1A and F1B generations. There was a significant (p < 0.01) decrease in the sex ratio of the F1A pups when compared with controls. Pup mortality was significantly (p < 0.01) increased in the F1B generation in comparison with controls, but the viability of the F2A and F2B pups was not affected. Effects were seen at doses above the threshold of saturation of renal clearance and at doses causing parental toxicity. No adverse effects on fertility were seen in males or females at any dose or in any generation (Rodwell, 1985).
Although morphological changes in male reproductive organs, increased follicle-stimulating hormone (FSH) and luteinizing hormone (LH) levels, and altered sperm number and motility were seen in male rats gavaged for 30 days with either100 or 200 mg/kg bw per day of 2,4-D, these effects occurred at doses that exceeded renal saturation (Marouani et al., 2017).
No evidence of developmental neurotoxicity was found in an Extended One-Generation Reproductive Toxicity Study (pre-OECD guideline 443) in which CD rats were fed 2,4-D (6–45 mg/kg bw per day) during critical windows of development (pre-mating, mating, gestation and lactation) (Marty et al., 2013). Other available neurotoxicity studies were unsuitable for risk assessment as they used only one or two doses; however, they may indicate a potential sensitivity of offspring to 2,4-D.
Pregnant Wistar rats were fed diets containing 70 mg/kg of 2,4-D from GD 16 to PND 23 (Bortolozzi et al., 1999). Weaned pups were divided into 2 groups with 1 group maintained on the 2,4-D diet until PND 90. Treated pups had delayed righting reflex and negative geotaxis as well as motor abnormalities and stereotypic behaviours. As adults, the treated pup group had serotonin syndrome behaviours, catalepsy and right-turning preference. Using the same protocol, Bortolozzi et al. (2003) showed that treated pups had altered monoamine systems in their brains.
In Duffard et al. (1996), oral exposure of pups through mother's milk (maternal dose of 100 mg/kg bw per day of 2,4-D via intraperitoneal injection) during PND 15 to 25 resulted in myelin deficiency in the central nervous system of pups. In De Duffard et al. (1995), pregnant Wistar rats received 0 or 70 mg/kg bw per day of 2,4-D via intraperitoneal injection from 9 to 25 days postpartum. Pups were exposed to 2,4-D via lactation and had changes in serotonin-immunoreactive neurons.
Dose- and time-dependent decreased body and brain weights and decreased protein and DNA levels were seen in neonate Wistar rats subcutaneously injected with either 70 or 100 mg/kg bw per day of 2,4-D on either PND 7–17, PND 7–25, PND 12–17 or PND 12–25 (Rosso et al., 1997). In a similar study using the same protocol, treated pups had lower myelin deposits and alterations in righting reflex, geotaxy, forelimbs support and open field testing (Rosso et al., 2000).
Strutz et al. (2000) studied the pups of dams that were fed diets containing 50, 70 or 100 mg/kg of 2,4-D during lactation. Residues of 2,4-D were found in the stomach contents, blood, brain and kidneys of 4-day-old pups. Residue levels were both dose- and exposure-time-dependent.
2.5 Genotoxicity and carcinogenicity
Negative results were obtained in the following in vitro studies: unscheduled DNA synthesis assay using rat hepatocytes, sister chromatid exchange (SCE) assays using Chinese hamster ovary cells, chromosomal aberration assays using human peripheral lymphocytes, apurinic/apyrimidine site activity assays using human fibroblasts and Ames assays using several strains of Salmonella typhimurium both with and without metabolic activation (Linnainmaa, 1984; Mustonen et al., 1986; Clausen et al., 1990; Charles et al., 1999a; Gollapudi et al., 1999).
Two in vitro studies were positive for genotoxic effects. Gonzalez et al. (2005) reported dose-dependent increases in SCE and DNA-strand breaks in Chinese hamster ovary cells in a Comet assay. However, the study had a number of limitations: a weak dose-response relationship with no apparent time dependence, limited concentration range, a lack of a positive control, and amalgamation of the untreated and vehicle control results (Gonzalez et al., 2005). A hypoxanthine-guanine phosphoribosyltransferase locus assay using V79 Chinese hamster fibroblast cells showed 2,4-D was positive for gene mutation but only at high doses that were also cytotoxic (Pavlica et al., 1991).
Negative results were seen in 2 in vivo studies: a sex-linked recessive lethal test and a screening study testing for chromosome breakage and loss in Drosophila melanogaster fed 2,4-D (Woodruff et al., 1983; Zimmering et al., 1985). A few positive results have been reported in Drosophila,but they were observed at high doses and in unstable strains (Munro et al., 1992; Kaya et al., 1999). No chromosomal abnormalities or DNA lesions were observed in hepatocytes, lymphocytes or bone marrow cells of rats, mice and Chinese hamsters given 2,4-D orally and tested using bone marrow micronuclei, unscheduled DNA synthesis, and SCE assays (Linnainmaa, 1984; Charles et al., 1999a, 1999b). A study by Amer and Aly (2001) did show chromosome aberrations in bone marrow and sperm head abnormalities in Swiss mice gavaged with 3.3 and 333 mg/kg bw of 2,4-D for 3 or 5 days; however, only 500 cells were counted and the increase in the percentage of abnormalities was quite small.
Although some studies have given positive results, the overall lack of genotoxicity following in vitro and in vivo exposure to 2,4-D is consistent with its characteristics as a weak acid that is not significantly metabolized and that is excreted rapidly from the body (Munro et al., 1992).
Neoplasms were not increased in a 2-year oncogenicity study where B6C3F1 mice were fed diets containing up to 300 mg/kg bw per day of 2,4-D (Jeffries et al., 1995; Charles et al., 1996a). Munro et al. (1992) describes an unpublished study in which the incidence of astrocytoma (brain cancer) was slightly increased in male rats given 45 mg/kg bw per day of 2,4-D; however, the characteristics of the tumours were atypical of a chemical carcinogen and repeat studies failed to confirm the results (Jeffries et al., 1995; Charles et al., 1996a; Kennepohl et al., 2010).
The International Agency for Research on Cancer (IARC) has classified 2,4-D in group 2B, "possibly carcinogenic to humans" based on limited evidence in animals; other organizations have used a non-cancer approach to assess risk to human health from this pesticide (Loomis et al., 2015; IARC, 2017). The US EPA (2005, 2007) has repeatedly described 2,4-D as not classifiable with regard to human carcinogenicity. The Joint FAO/WHO Meeting on Pesticide Residues concluded that the carcinogenic potential of 2,4-D could not be evaluated on the basis of the available epidemiological studies and that 2,4-D and its salts and esters were not genotoxic (WHO, 2017). Moreover, in its re-evaluation for the continuing registration of 2,4-D, Health Canada (2005a, 2007) concluded that 2,4-D is not carcinogenic, based on the absence of evidence of cancer in animals and on the lack of a clear association between exposure and cancer in human studies.
2.6 Mode of action
The kidney is the most sensitive target organ of 2,4-D toxicity (Gorzinski et al., 1987; Charles et al., 1996a, 1996b; Health Canada, 2007). Effects seen (increased relative and absolute kidney weights, changes in renal histopathology particularly in the proximal tubules, and alterations in clinical chemistry) are consistent across species tested in both subchronic and chronic studies and are related to saturation of the renal clearance mechanism (Serota, 1983a, 1983b; Gorzinski et al., 1987; Schultze, 1991a; Munro et al., 1992; Jeffries et al., 1995; Charles et al., 1996a, 1996b).
In the kidney, 2,4-D accumulates in the renal proximal tubules through the action of a metabolically active renal organic anion transporter, OAT1 (Hasegawa et al. 2003; Timchalk, 2004; Nozaki et al. 2007; Burns and Swaen, 2012; Saghir et al., 2013). The OAT1 transporter plays a critical role in the dose-dependent systemic renal clearance of 2,4-D in rats and is saturated at oral gavage and dietary doses of approximately 50 mg/kg bw in male Fischer 344 rats given a single dose of 2,4-D (Gorzinski et al., 1987; Saghir et al., 2013). Sprague-Dawley rats given daily doses of 2,4-D reached renal saturation at 63 mg/kg bw per day for males (dosed for 71 days) and at 14 to 27 mg/kg bw per day for females (dosed for 96 days) (Saghir et al., 2013).
The mode of action for 2,4-D has not been clearly established. An increase in oxidative stress represents the most compelling evidence for the toxic mode of action of 2,4-D in animals, and seems to be responsible for the alterations observed in the kidney (degeneration of the proximal tubules, vacuolization of tubular cells and loss of the brush border) (Bongiovanni et al., 2011; Wafa et al., 2011). 2,4-D has been shown to perturb cell metabolism, deplete glutathione reserves and thiol levels, and stimulate the peroxisome proliferator-activated receptor. These precursor effects lead to an increase in reactive oxygen species production that can induce kidney, liver and nervous system toxicity. It could also explain the genotoxic effects observed at high doses (Argese et al., 2005). An increase in markers of oxidation (hydroxyl radical, protein oxidation, carbonyl groups, lipid peroxides), a decrease in the glutathione:glutathione disulfide ratio and in protein thiol content have been observed in rats exposed orally to 2,4-D (Ferri et al., 2007; Nakbi et al., 2012; Tayeb et al., 2012; Pochettino et al., 2013). These alterations were associated with a decrease in kidney and hepatic antioxidant enzyme activities, such as superoxide dismutase, catalase, glutathione peroxidase and glutathione reductase in rats. Wistar rats administered 15–150 mg/kg bw of 2,4-D butylglycol by gavage had an increase in hepatic lipid peroxidation and a decrease in catalase, glutathione peroxidase and glutathione reductase at all doses, and superoxide dismutase at high doses after 4 weeks, supporting the oxidative stress mode of action (Tayeb et al., 2013).
Moreover, in vitro studies using human and rat kidney cell lines and hepatocytes support the generation of reactive oxygen species (Palmeira et al., 1995; Duchnowicz and Koter, 2003; Bharadwaj et al., 2005; Bukowska et al., 2008; Troudi et al., 2012).
2.7 Selected key study
2,4-D has been extensively studied and has a large and comprehensive toxicology database. During its toxicology re-evaluation of 2,4-D, PMRA critically examined the totality of the scientific database, including proprietary (unpublished) and published toxicity studies, and identified the kidney as the most sensitive target organ across the database (Health Canada, 2005a, 2007). Although no epidemiological studies have investigated the effects of 2,4-D on the kidney, kidney effects have been consistently observed in subchronic and chronic mice and rat studies (Serota et al, 1983a, 1983b; Serota, 1986; Gorzinski et al., 1987; Schultze, 1991; Jeffries et al., 1995; Charles et al., 1996a).
PMRA (Health Canada 2007, 2018a) identified two long-term rat studies (Serota, 1986; Jeffries et al., 1995) that showed kidney effects as the key studies and their NOAELs of 5 mg/kg bw per day as the point of departure (POD) for the human health risk assessment of 2,4-D and the derivation of an acceptable daily intake (ADI).
In the Serota (1986) study, F344 rats (60/sex/dose) fed diets containing 0, 1, 5, 15 or 45 mg/kg bw per day of 2,4-D for 2 years showed no effect on clinical chemistry, gross pathology or survival. Relative body weight gain in high-dose females was significantly reduced at 12 and 24 months, although food consumption was decreased at 12 months only. Absolute and relative kidney weights were increased in both males (≥ 15 mg/kg bw per day) and females (all doses) at 2 years. Renal pelvic mineralization was seen in females starting at 15 mg/kg bw per day. Changes in kidney histopathology (increased incidence of brown tubular pigment and severity of fine cytoplasmic vacuolization in the renal cortex) were seen in both sexes starting at 5 mg/kg bw per day. PMRA's 2005 assessment (Health Canada, 2005a) proposed a NOAEL of 1 mg/kg bw per day for Serota (1986) based on pigmentation changes. However, additional data submitted during the consultation period resulted in a reassessed NOAEL of 5 mg/kg bw/day for this study (Health Canada, 2005b). The new data included an analysis of kidney samples from the Serota (1986) study by an independent pathology working group. The pathology working group did not find any difference in tubular cell pigmentation between the dosed and control groups. It also concluded that the nature of the pigment in all study animals was morphologically similar to that known to occur spontaneously in F344 rats of this age. The incidence and severity of pelvic mineralization was considered to be treatment-related in males at 45 mg/kg bw per day and in females at 15 and 45 mg/kg bw per day resulting in a NOAEL of 5 mg/kg bw per day (Health Canada, 2005b, 2007).
Renal effects consisting of slightly altered clinical chemistry and kidney weights were seen in a 2-year chronic toxicity/oncogenicity study by Jeffries et al. (1995). In this study, F344 rats (60/sex/dose) were fed diets containing 0, 5, 75 or 150 mg/kg bw per day of technical grade 2,4-D (purity 96.4%) with an interim sacrifice of 10 rats/sex/dose at 12 months. Animal survival was unaffected by treatment. At 2 years, kidney weights were unaffected in males, whereas females had a slight, statistically significant decrease at 150 mg/kg bw per day and a statistically significant increase in kidney to body weight ratio at ≥ 75 mg/kg bw per day. Decreased food consumption was seen in females at ≥ 75 mg/kg bw per day and in males at 150 mg/kg bw per day; it was accompanied by decreased weight gain. At ≥ 75 mg/kg bw per day, BUN was decreased in males only, while creatinine was increased in females only. At interim sacrifice, both males and females dosed with ≥ 75 mg/kg bw per day had degeneration of the proximal convoluted tubules, but no effects on kidney histopathology were noted in rats sacrificed at 2 years (Jeffries et al., 1995). A NOAEL of 5 mg/kg bw/d was established based on kidney pathology (degeneration of descending proximal convoluted tubules) noted at the next dose level. Although degeneration of the descending proximal convoluted tubules was only seen at interim sacrifice and not at study termination, it was considered toxicologically significant as the kidney was identified across the database as the most sensitive target organ (Health Canada, 2007).
In addition, the selection of a NOAEL of 5 mg/kg bw per day as the POD for the determination of the ADI was supported by the NOAEL of 5 mg/kg bw per day established in the chronic mouse study in which kidney pathology (increased weight, degeneration with regeneration of descending limb of proximal tubule, decreased vacuolization and mineralization of proximal tubule, multifocal cortical cysts) occurred at doses ranging from 62 to 300 mg/kg bw per day (Jeffries et al., 1995; Charles et al., 1996a; Health Canada, 2007, 2018). Although this study was not selected to establish the ADI, the NOAEL value, the endpoint, and the duration of the study are all the same as those used for the ADI. Thus, this study further supported the POD used in establishing the ADI.
Kidney toxicity was observed at lower dose levels across the 2,4-D database compared to all other adverse effects, including sensitivity of the young. The study by Serota et al. (1986) employed five dose levels of 0, 1, 5, 15, and 45 mg/kg bw/day while the study by Jeffries et al. (1995) used four dose levels of 0, 5, 75, and 150 mg/kg bw/day. In both studies, kidney pathology was observed at a wide dose range of 15–150 mg/kg bw per day. In adult rats, neurotoxic effects (incoordination and slight gait abnormality) were evident after a single high-dose exposure but were resolved 4 days later (Mattsson et al., 1997). Repeated high dose exposure (150 mg/kg bw per day) also affected forelimb grip strength and induced retinal degeneration (Mattsson et al., 1997). Published studies using 1 or 2 doses and involving intraperitoneal administration of 100 mg/kg bw per day of 2,4-D acid to pregnant rats, subcutaneous administration of 70 or 100 mg/kg bw per day to pups as well as studies focusing on oral exposure of pups through mother's milk (maternal doses 100 mg/kg bw per day) during PND 15 to 25, resulted in myelin deficiency in the central nervous system of pups (De Duffard et al., 1995; Duffard et al., 1996; Rosso et al. 1997, 2000). These studies were unsuitable for risk assessment but may indicate a sensitivity of the young to 2,4-D. Another study using a combination of prenatal and postnatal exposures (70 mg/kg bw per day of 2,4-D in diet of dams and pups) showed a delay in the development of the surface righting reflex, geotaxic response and hindlimb support in rat pups, which correlated with alterations in the development of the monoamine systems in the brains of these rats as adults (Bortolozzi et al. 1999, 2003). Residues of 2,4-D were also found in the brains of pups of treated dams (Sturtz et al. 2000). Although these effects were observed at much higher dose levels relative to the doses causing the primary target effects (i.e., kidney toxicity) in the short- and long-term studies, these findings may be an indication of offspring sensitivity after exposure to 2,4-D during prenatal and postnatal development. Potential sensitivity of the young was also noted in the limited reproductive toxicity study (Rodwell, 1985), which was considered deficient in some respects, including excess mortality at 80 mg/kg bw per day resulting in that dose being dropped after the first generation because it exceeded the maximum tolerable dose, misdosing of F1B pups resulting in excessive mortality and no dose concentration adjustment (Neal et al., 2017).
3.0 Derivation of the human health reference value
As noted above, the NOAEL of 5 mg/kg bw per day for kidney effects in rats was selected as the basis for the current risk assessment. In deriving the ADI, PMRA applied a 300-fold uncertainty/safety factor which included standard uncertainty factors of 10-fold for interspecies extrapolation and 10-fold for intraspecies variability as well as an additional 3-fold uncertainty factor to account for potential sensitivity in the young noted in the limited reproductive toxicity study by Rodwell (1985) and in a series of published neurotoxicity studies (Health Canada, 2007). The reproductive toxicity study was considered deficient in some respects and the published neurotoxicity studies identified sensitivity of the young beginning at dose levels of 20 mg/kg bw/day and 60 mg/kg bw/day, respectively. These NOAELs were higher than the NOAEL used to establish the ADI and the 2,4-D toxicity database included extensive assessment of potential effects in the young. However, given the lack of acceptable OECD guideline compliant multigenerational reproductive toxicity and developmental neurotoxicity studies examining potential effects in the young at that time; both of which are typically required in these scenarios for pesticide registrations/re-evaluations, the application of a 3-fold uncertainty factor was considered justified in the PACR2005-01 and PACR2007-06 (Health Canada, 2005a, 2007).
An extended one-generation reproductive toxicity study by Marty et al. (2013) was submitted to PMRA to address these data gaps after the publication of the RVD2008-11 document (Health Canada, 2008, 2013). PMRA established a NOAEL of 21 mg/kg bw/day for offspring toxicity in the Marty et al. (2013) study. Since the NOAEL of 5 mg/kg bw/day used to derive the ADI was lower than the NOAEL of 21 mg/kg bw/day for offspring toxicity in this study, revisiting the choice of the POD for the ADI was not deemed necessary and further supported the fact that the 3-fold uncertainty factor that had been applied in the original assessment was protective of potential effects in the young. The ADI (Health Canada, 2007, 2018b) was calculated as follows:
Equation 1
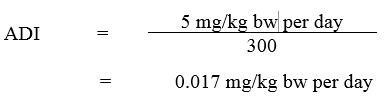
Equation 1 - Text Description
The ADI for 2,4-D is 0.017 mg/kg bw per day. This is calculated by dividing the NOAEL of 5 mg/kg bw per day by the uncertainty factor of 300.
where:
- 5 mg/kg bw per day is the NOAEL, based on kidney effects; and
- 300 is the uncertainty factor, selected to account for interspecies variation (×10), intraspecies variation (×10), and potential sensitivity to the young, noted in a limited rat reproduction study and in a series of published neurotoxicity studies (×3).
Based on the ADI of 0.017 mg/kg bw per day, a health-based value (HBV) for 2,4-D in drinking water was derived as follows:
Equation 2
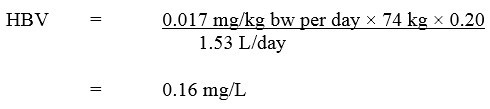
Equation 2 - Text Description
The HBV for 2,4-D in drinking water is 0.16 mg/L. This is calculated by multiplying the ADI for 2,4-D (0.017 mg/kg bw per day) by the allocation factor for water (0.2), then by the average body weight for an adult (74 kg). This product is then divided by the daily volume of water consumed by an adult (1.53 L/day).
where:
- 0.017 mg/kg bw per day is the ADI calculated using a NOAEL of 5 mg/kg bw/day (Health Canada, 2007);
- 74 kg is the adult body weight (Health Canada, 2021);
- 1.53 L per day is the daily volume of tap water consumed by an adult (Health Canada, 2021);
- 0.20 is the default allocation factor for drinking water, since drinking water is not a major source of exposure to 2,4-D and there is evidence of the presence of 2,4-D in one of the other media (i.e., food) (Krishnan and Carrier, 2013).
4.0 Analytical and treatment considerations
4.1 Analytical methods to detect 2,4-D
Standardized methods available for the analysis of 2,4-D in source and drinking water and their respective method detection limits (MDL) are summarized in Table 2. MDLs are dependent on the sample matrix, instrumentation, and selected operating conditions and will vary between individual laboratories. The MDLs or method reporting limits from provincial and territorial data are in the range of 0.005 to 1.0 μg/L (Ministère du Développement Durable, de l'Environnement, de la Faune et des Parcs, 2019; Nova Scotia Environment, 2019; PEI Department of Communities, Land and Environment, 2019).
Drinking water utilities should discuss sampling requirements with the accredited laboratory conducting the analysis to ensure that quality control procedures are met and that method reporting limits are low enough to ensure accurate monitoring at concentrations below the maximum acceptable concentration (MAC). Sample processing considerations for the analysis of 2,4-D in drinking water (e.g. sample preservation, storage) can be found in the references listed in Table 2. In addition, Clausen (2000) reported that 2,4-D was retained on filters made of cellulose acetate, nylon or polyethersulfone materials when sampling or analyses involved filtering the water sample to separate suspended solids from solution. Filters made of polyvinylidene fluoride or polytetrafluorethylene materials were found to retain less 2,4-D.
Method | Technique | MDL (µg/L) |
Interferences |
---|---|---|---|
EPA 515.1 revision 4.1 (US EPA, 1995a) |
Gas chromatography with electron capture detector (GC/ECD) | 0.078 | Alkaline substances; organic acids and phenols; plastic; analyzing a low concentration sample immediately after a high concentration sample |
EPA 515.2 revision 1.1 (US EPA, 1995b) |
Liquid–solid extraction (LSE) and GC/ECD | 0.28 | Alkaline substances; organic acids and phenols; plastic/phthalates |
EPA 515.3 revision 1.0 (US EPA, 1996) |
Liquid–liquid extraction, derivatization and GC/ECD | 0.35Footnote a to 0.36Footnote b | Analyzing a low concentration sample immediately after a high concentration sample; plastic/ phthalates; presence of water from base promoted esterification procedure |
EPA 515.4 revision 1.0 (US EPA, 2000) |
Liquid–liquid microextraction, derivatization and GC/ECD | 0.055 to 0.066Footnote c | Plastic/phthalates; sodium sulphate |
EPA 555 revision 1.0 (US EPA, 1992) |
High performance liquid chromatography with photodiode array ultraviolet detector (HPLC/PDA-UV) | 0.34 to 1.3Footnote c | Sodium sulphate |
ASTM D5317-98 (Reapproved 2011) (ASTM, 2011) |
GC/ECD | 0.2 | Alkaline substances; organic acids and phenols; phthalate esters (e.g., flexible plastics); analyzing a low concentration sample immediately after a high concentration sample |
Standard Method 6640B or online version 6640B-01 (APHA, 2005, 2012, 2017) |
Liquid–liquid microextraction, derivatization and GC/ECD | 0.06 to 0.07Footnote c | Phthalate esters; avoid the use of plastics in the laboratory |
GC/ECD – gas chromatography/electron capture detector
|
4.2 Treatment considerations
Treatment technologies are available to effectively decrease 2,4-D concentrations in drinking water. Activated carbon adsorption is recognized as the best available technology for 2,4-D removal (US EPA, 2009b). Biological filtration processes can also decrease 2,4-D concentrations; however, conventional treatment is not effective for 2,4-D removal. Typical disinfection processes used in drinking water treatment also have limited potential to reduce 2,4-D concentrations. At the residential scale, certified treatment devices are available for the removal of 2,4-D. These devices rely mainly on adsorption (activated carbon) and reverse osmosis technologies.
4.2.1 Municipal-scale treatment
Since 2,4-D concentrations are low in source water, treatment technology data reported in the literature generally have low influent concentrations (< 10 µg/L). Information on the removal efficiencies and operational conditions from these studies is reported below, as the studies provide an indication of the effectiveness of specific treatment technologies for 2,4-D removal. The selection of an appropriate treatment process for a specific water supply will depend on many factors, including the raw water source and its characteristics, the operational conditions of the selected treatment method and the utility's treatment goals.
4.2.1.1 Conventional treatment
Conventional drinking water treatment processes, such as chemical coagulation, clarification, rapid sand filtration and chlorination, are reported to be ineffective in decreasing the concentration of a variety of classes of pesticides, including polar pesticides like phenoxyacetic acids (Robeck et al., 1965; Miltner et al., 1989; Croll et al., 1992; Haist-Gulde et al., 1993; Frick and Dalton, 2005; Chowdhury et al., 2010; Hughes and Younker, 2011). Biological filtration (see section 4.2.1.4) has shown some capacity for removing chlorophenoxy acids (Foster et al., 1991, 1992) and 2,4-D specifically (Woudneh et al., 1996, 1997; Storck et al., 2010; Zearley and Summers, 2012, 2015; Huntscha et al., 2013).
4.2.1.2 Oxidation and hydrolysis
Chamberlain et al. (2012) conducted bench-scale tests to assess what degradation of pesticides could be expected to occur naturally in the environment by hydrolysis/photolysis and by disinfection processes typically used in drinking water treatment. Common oxidation/ disinfection processes were evaluated as outlined in Table 3. Laboratory experiments were conducted at 23 ± 1 °C at pHs of 6.6 and 8.6 and an initial 2,4-D concentration of 25 µg/L. Solutions were spiked with an oxidant or subjected to UV photolysis at 254 nm at the doses noted in Table 3. Reaction media for the hydrolysis experiments were sodium-phosphate-buffered laboratory water at pHs 2, 7 and 12. Removal of 2,4-D was low in all experiments.
The ozone (O3) findings in Table 3 are consistent with predicted removal based on O3 rate constant studies for 2,4-D at typical drinking water exposures (Yao and Haag, 1991; Xiong and Graham, 1992; Hu et al., 2000; Benitez et al., 2004; Giri et al., 2007). Meijers et al. (1995) reported similar low reductions of 2,4-D using typical O3 dosages (reported as the O3 to dissolved organic carbon [DOC] ratio) in bench-scale experiments at 5 °C (pH 7.2, O3:DOC = 0.53, 2,4-D influent concentrations = 0.9–6.4 µg/L). However, 2,4-D removal increased to 48% at 20 °C (pH 7.2, O3:DOC = 0.55) and 74% when the pH and O3 dose were increased (pH 8.3, O3:DOC = 0.95).
Process | Dose used | CTFootnote a Range (mg·min/L) | Decrease (%) |
---|---|---|---|
Free chlorine | 2–5 mg/L | 107–173 | < 20 |
Monochloramine | 9–14 mg/L | 1287–1430 | <–20 |
Permanganate | 3–5 mg/L | 134–164 | <–20 |
Chlorine dioxide | 2–3 mg/L | 38–73 | <–20 |
Hydrogen peroxide | 100 mg/L | 933–1100 | <–20 |
Ozone | 1–2 mg/L | 0.2–0.3 | <–20 |
UV254 | 77–97 mV·s/cm2 | <–20 | |
Source: Chamberlain et al. (2012) |
The UV findings in Table 3 are also consistent with pilot-plant experiments conducted by Kruithof et al. (2002) and bench-scale experiments conducted by Benitez et al. (2004). Kruithof et al. (2002) reported a 58% decrease in 2,4-D at a UV dose of 2000 mJ/cm2, much higher than needed for disinfection. Benitez et al. (2004) reported a decrease of 30%–40% following 100 minutes of reaction time at pHs 9 and 7, respectively (initial 2,4-D concentration of 50 mg/L) using a low-pressure UV lamp.
4.2.1.3 Adsorption
Activated carbon adsorption is a widely used technology to reduce the concentration of micropollutants in drinking water (Haist-Gulde and Happel, 2012; van der Aa et al., 2012). Activated carbon can be applied in two ways: slurry applications using powdered activated carbon (PAC) or fixed-bed reactors with granular activated carbon (GAC) (Chowdhury et al., 2013).
Powdered activated carbon
Many pesticides have been found to strongly adsorb to PAC (Chowdhury et al., 2013). The use of PAC offers the advantage of providing virgin carbon when required (e.g., during the pesticide application season) (Miltner et al., 1989). The capacity of PAC to remove pesticides by adsorption depends on the PAC dose, the contact time, the PAC characteristics (type, particle size), the adsorbability of the contaminant and the competition for adsorption sites from natural organic matter (NOM) (Haist-Gulde and Happel, 2012).
Although there is a lack of published literature regarding the use of PAC in drinking water treatment specifically for 2,4-D adsorption, based on its chemical properties and published research on adsorption technology, PAC is expected to adequately remove 2,4-D (Haist-Gulde, 2014). As removal efficiency varies by PAC type, adsorption kinetics should be considered when optimizing PAC systems (Gustafson et al., 2003; Summers et al., 2010). Jar tests, with varied carbons and conditions, are generally used to develop percentage-removal-versus-PAC-dosage curves.
Increasing the PAC dose will increase the removal percentage for a contaminant but not in a proportional ratio (Robeck, 1965; Miltner et al., 1989; Gustafson et al., 2003; Westerhoff et al., 2005; Chowdhury et al., 2013). Increasing the contact time will increase the amount of pesticide removed, as the time to equilibrium (i.e., contact time needed to utilize the full capacity of the PAC) is generally greater than that used by water treatment plants (Gustafson et al., 2003). Decreasing the PAC size reduces the time to equilibrium. However, the benefit from decreasing the PAC size is typically offset by the difficulty in removing the smaller PAC from solution (Chowdhury et al., 2013).
Generally, the presence of NOM adds complexity to PAC treatment because the NOM competes directly for adsorption sites or fouls the PAC by blocking pores (Chowdhury et al., 2013). Robeck et al. (1965) found that a PAC dose of 29 ppm was required to reduce lindane concentrations from 10 ppb to 1 ppb in river water (chemical oxygen demand: 5–35 ppm) compared with a dose of 2 ppm in distilled water. This was attributed to the NOM found in the river water. Responsible authorities should be aware of the impact of NOM on PAC systems, as it may impact water quality objectives for 2,4-D removal. In addition, PAC dosage for taste and odour control, a common application of PAC, may be a fraction of that required for 2,4-D removal.
When PAC is added prior to some treatment processes, it can interact with water treatment chemicals, leaving less PAC available to lower concentrations of contaminants such as 2,4-D (Greene et al., 1994; Summers et al., 2010). Kouras et al. (1998) reported that the addition of PAC before coagulant dosing increased the required PAC dose from 20 mg/L to 40 mg/L to reach a target concentration of 0.1 µg/L for lindane. This was attributed to the PAC particles becoming incorporated into the floc structure, thereby reducing available adsorption sites.
Granular activated carbon
The use of GAC is an effective approach for treating organic contaminants that are regularly found in source water at concentrations of concern (Chowdhury et al., 2013). According to the WHO (2011), GAC treatment should be able to decrease 2,4-D concentrations to 1 µg/L. The capacity of GAC to remove pesticides by adsorption depends on the filter velocity, the empty bed contact time (EBCT), the GAC characteristics (type, particle size, reactivation method), the adsorbability of the contaminant, the filter run time and the competition from NOM for adsorption sites (Haist-Gulde and Happel, 2012).
A pilot-scale study demonstrated the capacity of GAC to remove 2,4-D (Haist-Gulde and Happel, 2012). Three GAC fixed-bed adsorbers were installed in parallel at a surface water treatment plant and each was filled with a different bituminous coal carbon. The pilot plant was operated for 21 months under typical conditions, and breakthrough curves were measured for ionic pesticides and metabolites, including 2,4-D. Feed water was taken from the GAC filter influent for the full-scale surface water treatment plant (DOC = 1.3 mg/L) and spiked with 1 µg/L of 2,4-D. The breakthrough of 2,4-D was measured at bed depths of 0.5 m, 1.0 m, and 1.5 m, corresponding to EBCTs of 3 min, 6 min, and 9 min, respectively, at a filter velocity of 10 m/h. Complete removal was possible to about 50,000 bed volumes (BVs) at a 1.5 m bed depth, although the performance of the three carbons varied (Haist-Gulde and Happel, 2012). Reported BVs treated to 10% breakthrough were 37 000, 56 000 and 73 000 (at a 1.5 m bed depth) for carbons 1, 2 and 3, respectively. The impact of bed depth was also evaluated; the operation time to 20% breakthrough ranged from approximately 30 000 to 80 000 BVs for bed depths of 0.5 m and 1.5 m, respectively. Performance at a 1.0 m bed depth was typically found to be slightly better than at 0.5 m. The study concluded that 2,4-D should be removed by most GAC adsorbers; short GAC bed depths are not suitable for the removal of ionic pesticides such as 2,4-D and GAC selection pilot studies are required (Haist-Gulde and Happel, 2012).
Because GAC fixed-bed adsorbers are typically operated on a continuous basis, NOM adsorbs on the GAC during periods when contaminants such as 2,4-D are absent from the source water. The GAC becomes fouled (or preloaded) with NOM and the rate of adsorption for targeted contaminants such as 2,4-D is adversely affected. If the GAC is exhausted, it may be completely or partially ineffective for 2,4-D removal (Knappe et al., 1999; Summers et al., 2010; Haist-Gulde and Happel, 2012; Chowdhury et al., 2013).
Responsible authorities should be aware of the impact of NOM on GAC systems, as it may impact water quality objectives for 2,4-D removal. DOC monitoring is recommended as a conservative tracer for contaminant breakthrough (Corwin and Summers, 2012; Kennedy et al., 2012).
4.2.1.4 Biological filtration
Biological filtration processes include slow sand filtration, riverbank filtration and engineered biological filtration. With slow sand filtration, the significant removal mechanism is biodegradation (Woudneh et al., 1997). With riverbank filtration, removal mechanisms include adsorption, biodegradation and transport processes such as convection, diffusion and dispersion (Storck et al., 2010). However, adsorption plays a minor role for polar organic compounds such as 2,4-D (Huntscha et al., 2013). Engineered biofiltration is a modification of conventional filtration whereby the filter media (i.e., anthracite/sand or GAC) has been allowed to develop a microbial biofilm that assists in the removal of fine particulate and dissolved organic materials (Symons et al., 2000). The filter media influences the removal mechanism, and both adsorption and biodegradation can occur when using GAC (van der Aa et al., 2012), whereas biodegradation occurs with anthracite/sand (Zearley and Summers, 2012, 2015). Although biological filtration has the ability to decrease 2,4-D concentrations, Benner et al. (2013) noted that the removal mechanisms remain largely unknown. The authors recommended more research to fill knowledge gaps and enable process optimization for the removal of micropollutants, including those that are less biodegradable and those that are present on an intermittent basis.
Slow sand filtration
A pilot-scale study conducted in the United Kingdom demonstrated the capacity of slow sand filtration to remove 2,4-D (Woudneh et al., 1996, 1997). This study dealt with both the seasonal variation (Woudneh et al., 1996) and the impact of filter maturity (Woudneh et al., 1997) on 2,4-D removal. In brief, two filter bed depths (300 mm and 500 mm) and two flow rates (0.06 m/h and 0.12 m/h) were studied over 15 months in three study periods: 1) October to December 1994, 2) March 1995, and 3) August and September 1995.
For the first study period, removals of approximately 90% were observed, as summarized in Table 4.
Filter bed depth (mm) | Flow rate (m3/h) | Contact time (h) | Removal efficiency (%) |
---|---|---|---|
300 | 0.06 | 4.3 | 89.6Footnote c |
0.12 | 2.15 | 90.5Footnote d | |
500 | 0.06 | 7.16 | 93.3Footnote c |
0.12 | 3.58 | 91.6Footnote d | |
|
In the second study period, the filter beds were cleaned, the sand was replaced and the percentage removal dropped to zero. After 20 days, adequate biological growth was restored to achieve complete removal of 2,4-D. The authors cautioned that re-establishing the biological growth within a filter bed depends on a number of factors, such as the chemical/microbiological water quality and environmental conditions. Thus, the time to restore filter performance for the removal of 2,4-D will be site specific (Woudneh et al., 1997).
During the third study period, the authors found 100% breakthrough of 2,4-D. Woudneh et al. (1996) hypothesized that the reduction in performance was due to either 1) a change in the aerobic nature of the filter bed, caused by decreased dissolved oxygen content of the water, or 2) a change in NOM, measured by a significant reduction in UV absorbance (at 254 nm) during August and September. Lower UV absorbance suggests the presence of more hydrophilic NOM, which can be easily consumed by microorganisms. The authors suggested that the presence of this nutrient source (e.g., hydrophilic NOM) caused the microorganisms to not biodegrade the 2,4-D. Complete removal of 2,4-D returned in October when the UV absorbance increased, suggesting the presence of more hydrophobic NOM. Hydrophobic NOM tends to be more recalcitrant, so 2,4-D becomes the preferred nutrient source for the microorganisms. Pilot testing is recommended to ensure that slow sand filtration will successfully treat a source water (Bellamy et al., 1985a, 1985b; Logsdon et al., 2002).
Riverbank filtration
Natural attenuation through riverbank filtration is one of the most basic and inexpensive methods of water treatment (Verstraeten and Heberer, 2002; Sørensen et al., 2006). Huntscha et al. (2013) conducted field tests to develop 2,4-D breakthrough curves for a riverbank filtration site with short travel times (a few days) and oxic groundwater conditions most of the time. In-situ microbial reaction rates were measured for nine micropollutants, including 2,4-D. The test was conducted at >16°C with an initial 2,4-D concentration of 100 ng/L. First-order rate constants for 2,4-D degradation ranged from 0.1/h to 1.3/h, corresponding to a half-life of 0.5-6.7 h for 2,4-D. The results from two other riverbank filtration field studies with suboxic and anoxic groundwater conditions are summarized in Table 5. High removal efficiencies were observed at both sites. Chemical structure and physiochemical properties of a compound, retention time and redox setting are the key factors controlling the removal of a micropollutant (Storck et al., 2012).
Initial concentration (µg/L) |
Redox milieu | Residence time (Days) |
2,4-D removal efficiency (%) | Dilution (%) |
---|---|---|---|---|
0.095 | Suboxic | < 1–3 | 86 | None |
0.3 | Anoxic | 14 | > 97 | 20 |
|
Engineered biological filtration
Two bench-scale studies have demonstrated the capacity of engineered biofilters to remove 2,4-D (Zearley and Summers, 2012, 2015). In both studies, biofilters were constructed using biologically active sand, taken from a full-scale drinking water treatment plant where the source is impacted by upstream wastewater discharges and agricultural/urban runoff. The sand media had an effective size of 0.45 mm and an approximate uniformity coefficient of 1.3. Feedwater was dechlorinated municipal water (pH = 7.7, alkalinity = 40 mg/L as CaCO3), supplemented with dissolved organic matter to a target total organic carbon concentration of 3 mg/L. Removal rates in the order of 30%–49% were observed in both studies during the acclimation periods (100–159 days).
In the first study, the long-term removal of 2,4-D under different EBCTs was assessed. The study was conducted at lab temperature (20 ± 2 °C) and consisted of 2 columns in series operated at a hydraulic loading rate of 2.4 m/h to achieve a target EBCT of 7.5 min in the top column and 7.5 min in the bottom column (total ~ 15 min). The influent was spiked with 13 micropollutants (2,4-D influent concentration of 171 ± 57 ng/L) and operated for 350 days. Steady-state removal of 2,4-D was reported to be 68% ± 11% at an EBCT of 7.9 min and 77% ± 13% at an EBCT of 15.8 min (Zearley and Summers, 2012).
In the second study, 7 biofilters were used to assess the effects of various intermittent loading conditions (i.e., periods with no 2,4-D spiking). The target hydraulic loading rate of the biofilters was 2.2 m/h to achieve an EBCT of 8.7 min with an influent 2,4-D spike of 87 ng/L ± 7.6 ng/L. In the control biofilter (constant 2,4-D loading), a steady-state removal of 70% ± 3.8% at an average EBCT of 8.2 min was reported. For the remaining 6 biofilters, 2,4-D spiking was initially conducted for 60–96 days, followed by periods of no spiking for 5 different time periods: 0, 36, 83, 149, and 263 days. Biomass acclimation was observed in the intermittently loaded biofilters with removal rates of 35%–55%. Following 209 days of biofilter operation, greater than 70% removal was reported (regardless of no spiking for a period of 149 days), indicating that constant exposure to 2,4-D was not necessary for biomass acclimation. Adapted microorganisms were capable of biodegrading 2,4-D following non-exposure periods of up to 5 months; however, following 9 months of non-exposure, 2,4-D removal was significantly lower (approximately 20%) (Zearley and Summers, 2015).
4.2.1.5 Membrane filtration
Limited scientific literature has been published on the effectiveness of membrane treatment for the removal of 2,4-D from drinking water. Edwards and Schubert (1974) conducted bench-scale experiments to evaluate the removal rate of 2,4-D from an aqueous solution using cellulose acetate reverse osmosis membranes at a pressure of 50 psi. The rejection of 2,4-D from 10 mL samples initially varied between 52% and 65% but declined rapidly with successive 10 mL samples. Plakas and Karabelas (2012) reviewed the removal of a variety of pesticides from water by nanofiltration and reverse osmosis membranes. Although 2,4-D was not studied specifically, rejection rates varied from > 10% to 100%, depending on the pesticide and the membrane. More hydrophobic and polar pesticides, such as 2,4-D, tend to have lower rejection due to adsorption and diffusion through the membrane, as well as polar interactions with charged membranes.
4.2.1.6 Combined treatment processes
Donald et al. (2007) investigated the occurrence of 45 pesticides in 15 untreated surface water reservoirs in Manitoba, Saskatchewan and Alberta from May 2003 to April 2004. The authors subsequently evaluated the effectiveness of treatment associated with the reservoirs in July 2004 and July 2005. Mean concentrations for 2,4-D in untreated water ranged from 12 ng/L ± 6 ng/L to 597 ng/L ± 199 ng/L. The overall mean for all sites was reported as 123 ng/L (n = 163). In July 2004 and July 2005, 28 simultaneous raw and treated water samples were collected to assess pesticide reduction by the water treatment plants associated with each surface water reservoir. Treatment processes varied at the 15 sites and included both conventional and advanced processes. Thirteen facilities had sand filtration, 1 had membrane filtration and 1 had no filtration. All facilities had chlorination, with 2 facilities also adding ammonia for chloramination; 8 facilities used potassium permanganate, 3 facilities used lime-soda ash and carbon dioxide, and 12 facilities used some form of activated carbon. The authors reported a 39% mean reduction in 2,4-D concentrations (data not provided). The authors concluded that the decrease in concentrations for the 29 pesticides that were detected during the study period (including 2,4-D) was highly variable and showed no obvious relation to differences in treatment processes. As the study design was not intended to characterize treatment efficacy, it is unknown which treatment processes contributed to the reduction in 2,4-D concentrations reported in this study.
4.2.1.7 Advanced oxidation processes
Advanced oxidation processes (AOPs) use combinations of oxidants, UV irradiation and catalysts to generate hydroxyl free radicals in water to make oxidation-reduction reactions more rapid or complete (Foster et al., 1991; Symons et al., 2000).
Ozone and hydrogen peroxide: Limited scientific literature has been published on the effectiveness of O3 and hydrogen peroxide (O3/H2O2) for the removal of 2,4-D from drinking water. Meijers et al. (1995) conducted bench-scale experiments and found that 2,4-D was degraded by 88%–95% when ozonation was preceded by H2O2 dosage (O3/DOC = 1.4; H2O2/O3 = 0.5; pH 7.2–8.3; temp = 20ºC); pH was shown to have a minor effect on pesticide degradation using AOPs.
UV and hydrogen peroxide: Wols and Hofman-Caris (2012) reviewed the photochemical reaction constants required for UV AOPs and predicted low reduction for 2,4-D (~30%) using low-pressure UV lamps (400 mJ/cm2). Benitez et al. (2004) found that a combined UV/H2O2 process increased the rate of 2,4-D removal compared with UV treatment alone in bench-scale experiments. However, 20–40 min were required to achieve 80% reduction. Initial concentrations were high (50 mg/L) and the results did not include information on the final treated levels. Alfano et al. (2001) conducted bench-scale experiments (initial 2,4-D concentration = 30 mg/L) and found that a combined UV/H2O2 process was 20 times more effective than UV treatment alone.
4.2.2 Residential-scale treatment
In cases where 2,4-D removal is desired at the household level, for example when a household obtains its drinking water from a private well, a residential drinking water treatment unit may be an option for decreasing 2,4-D concentrations in drinking water. Before a treatment unit is installed, the water should be tested to determine the general water chemistry and 2,4-D concentration in the source water. Periodic testing by an accredited laboratory should be conducted on both the water entering the treatment unit and the treated water, to verify that the treatment unit is effective. Units can lose removal capacity through use and time and need to be maintained and/or replaced. Consumers should verify the expected longevity of the components in the treatment unit according to the manufacturer's recommendations and service it when required.
Health Canada does not recommend specific brands of drinking water treatment units, but it strongly recommends that consumers use units that have been certified by an accredited certification body as meeting the appropriate NSF International Standard/American National Standard Institute (NSF/ANSI) for drinking water treatment units. These standards ensure the material safety and performance of products that come into contact with drinking water. Certification organizations provide assurance that a product conforms to applicable standards and must be accredited by the Standards Council of Canada (SCC). Accredited organizations in Canada (SCC, 2019) include:
- CSA Group (www.csagroup.org);
- NSF International (www.nsf.org);
- Water Quality Association (www.wqa.org);
- UL LLC (www.ul.com);
- Bureau de Normalisation du Québec (www.bnq.qc.ca);
- Truesdail Laboratories Inc. (www.truesdail.com); and
- International Association of Plumbing and Mechanical Officials (www.iapmo.org)
An up-to-date list of accredited certification organizations can be obtained from the SCC (www.scc.ca).
A number of certified residential treatment devices are currently available for the removal of 2,4-D from drinking water. These devices rely on adsorption (activated carbon) and reverse osmosis technologies. Treatment devices to remove 2,4-D from untreated water (such as a private well) can be certified either specifically for 2,4-D removal or for the removal of volatile organic chemicals (VOCs) as a group by using a surrogate which addresses VOCs and other chemicals, including 2,4-D. Drinking water treatment devices can be installed at the faucet (point-of-use) or at the location where water enters the home (point-of-entry) in residential settings to reduce contaminant concentrations.
For a drinking water treatment device to be certified to NSF/ANSI Standard 53 (Drinking Water Treatment Units-Health Effects) for 2,4-D removal, it must be capable of decreasing an average influent concentration of 0.210 mg/L to a maximum final (effluent) concentration of 0.07 mg/L. For a drinking water treatment device to be certified to the same standard using the VOC surrogate, the device must be capable of removing 2,4-D from an average influent concentration of 0.110 mg/L by greater than 98% to a maximum final (effluent) concentration of 0.0017 mg/L (NSF/ANSI, 2018a).
For a drinking water treatment device to be certified to NSF/ANSI Standard 58 (Reverse Osmosis Drinking Water Treatment Systems) for the removal of VOCs, the device must be capable of reducing 2,4-D from an average influent concentration of 0.110 mg/L by greater than 98% to a final (effluent) concentration of 0.0017 mg/L (NSF/ANSI, 2018b). Water that has been treated using reverse osmosis may be corrosive to internal plumbing components. Therefore, these units should be installed only at the point-of-use. Also, as large quantities of influent water are needed to obtain the required volume of treated water, these units are generally not practical for point-of-entry installation.
5.0 Management strategies
All water utilities should implement a risk management approach, such as the source-to-tap or water safety plan approach, to ensure water safety (CCME, 2004; WHO, 2011, 2012). These approaches require a system assessment to characterize the source water, describe the treatment barriers that prevent or reduce contamination, identify the conditions that can result in contamination, and implement control measures. Operational monitoring is then established, and operational/management protocols are instituted (e.g., standard operating procedures, corrective actions and incident responses). Compliance monitoring is determined and other protocols to validate the water safety plan are implemented (e.g., record keeping, consumer satisfaction). Operator training is also required to ensure the effectiveness of the water safety plan at all times (Smeets et al., 2009).
5.1 Monitoring
2,4-D can be present in groundwater and surface water in areas where it is being used depending on the type and extent of its application, environmental factors (e.g., amount of precipitation, soil type, hydrogeological setting, etc.) and environmental fate (e.g., mobility, leaching potential, degradation, etc.) in the surrounding area. Water utilities should consider the potential for 2,4-D to enter source water (e.g., raw water supply to the drinking water system) based on site-specific considerations.
When it is determined that 2,4-D may be present and monitoring is necessary then surface and groundwater sources should be characterized to determine the concentration of 2,4-D. This should include monitoring of surface water sources during periods of peak use and rainfall events and/or monitoring of groundwater annually. Where baseline data indicate that 2,4-D is not present in source water, monitoring may be reduced.
Where treatment is required to remove 2,4-D, operational monitoring should be implemented to confirm whether the treatment process is functioning as required. The frequency of operational monitoring will depend on the water quality, fluctuations of the raw water concentrations and the treatment process. Responsible authorities should be aware of the impact of natural organic matter on activated carbon systems, as it may impact water quality objectives for 2,4-D removal.
Where treatment is in place for 2,4-D removal, compliance monitoring (i.e., paired samples of source and treated water to confirm the efficacy of treatment) should be conducted at a minimum, on an annual basis and during periods of peak use. When routine operational monitoring indicates the potential for contaminant breakthrough, such as with GAC, monitoring should be conducted quarterly to plan for the regeneration or replacement of the media.
6.0 International Considerations
This section presents drinking water guidelines, standards and/or guidance from other national and international organizations. Variations in these values can be attributed to the age of the assessments or to differing policies and approaches, including the choice of key study and the use of different consumption rates, body weights and source allocation factors (Table 6).
The US EPA (1991, 2005, 2010) established a drinking water maximum contaminant level (MCL) and a maximum contaminant level goal (MCLG) at 0.07 mg/L (70 µg/L) based on a reference dose (RfD) of 0.005 mg/kg bw per day and hematological, renal, and hepatic effects in subchronic and chronic rat studies, using an uncertainty factor of 100. The US EPA has concluded that 2,4-D is unclassifiable with respect to carcinogenicity in humans, based on inadequate data from epidemiological studies and the lack of adequate animal studies.
The WHO (2017) established a guideline value of 0.03 mg/L in 1998, based on an ADI of 0.01 mg/kg bw for the sum of 2,4-D and its salts and esters, expressed as 2,4-D. The ADI was based on a NOAEL of 1 mg/kg of body weight per day established in both a 1-year study of toxicity in dogs (based on serum chemical parameters and histopathological lesions in the liver and kidney) and a 2-year study in rats (based on kidney effects and using an uncertainty factor of 100) (JMPR, 1996; WHO, 2003).
The Australian drinking water guideline of 0.03 mg/L for 2,4-D was first established in 1989 and reconfirmed in 2006. It is based on a no-observed-effect level (NOEL) of 1 mg/kg bw per day for kidney effects in a 2-year rat study, using an uncertainty factor of 100 (NHMRC, NRMMC, 2011).
The European Union (EU) does not have a specific chemical parametric value for individual pesticides. Instead, the EU has a value of 0.1 µg/L for any individual (single) pesticide, and a value of 0.5 µg/L for total pesticides found in drinking water. In establishing these values, the EU did not consider the science related to each pesticide, such as health effects. Instead, the values are based on a policy decision to keep pesticides out of drinking water (EU, 2020).
Agency (Year) |
Value (mg/L) |
Key Endpoint (Reference) |
NOAEL /NOEL (mg/kg bw/d) |
UF | ADI (mg/kg bw/d) |
BW (kg) |
DW Intake (L/d) |
AF (%) |
Comments |
---|---|---|---|---|---|---|---|---|---|
HC - MAC (2021) |
0.10 | Kidney effects in rats and mice (Serota, 1986; Jeffries et al., 1995; Health Canada, 2018b) | 5* | 300 | 0.017 | 74 | 1.53 | 20 | HBV was calculated as 0.16 mg/L. *The NOAEL for the Serota study (1986) was previously set at 1 mg/kg/d but was re-assessed by a pathology working group in 2005 and increased to 5 mg/kg/d (see Section 2.4) |
US EPA (1991) |
0.07 | Kidney and liver effects in rats (Serota et al., 1983a) | 1 | 100 | 0.01 | 70 | 2 | 20 | Serota et al. (1983a) is the interim report for the Serota (1986) study used as the basis of the HC MAC. |
WHO (1998) |
0.03 | Kidney and liver effects in dogs and kidney effects in rats (JMPR, 1996) | 1 | 100 | 0.01 | 60 | 2 | 10 | JMPR (1996) uses a 1-year dog study (Dalgard, 1993) and a 2-year (unrevised*) rat study (Serota, 1986) as the point of departure. |
Australia (2011) |
0.03 | Kidney effects in rats | 1 | 100 | 0.01 | 70 | 2 | 10 | No reference is provided for the study (NHMRC and NRMMC, 2011). |
EU (2020) |
0.1 µg/L | The EU has a value of 0.1 µg/L for any individual (single) pesticide, and a value of 0.5 µg/L for total pesticides found in drinking water. In establishing these values, the EU did not consider the science related to each pesticide, including health effects. Instead, the values are based on a policy decision to keep pesticides out of drinking water. |
|||||||
ADI – Acceptable daily intake; |
7.0 Rationale
2,4-D is registered in Canada for use on turf, forests and woodlots, terrestrial feed and food crops, and industrial and domestic non-food sites. It is among the top 10 pesticides sold in Canada. Despite its common use in Canada, exposure data do not indicate significant levels in drinking water (provincial and territorial data show that levels in drinking water are generally below 1 µg/L). Although the IARC classified 2,4-D in group 2B, as possibly carcinogenic to humans (based on limited evidence in animals), international drinking water agencies have assessed 2,4-D based on its non-cancer effects. The kidneys are considered to be the target for 2,4-D's toxicity. Although no studies have investigated the effects of 2,4-D on the kidney in humans, kidney effects have been consistently observed in mice and rat studies. An HBV of 0.16 mg/L has been calculated based on kidney effects in mice and rats.
Health Canada in collaboration with the Federal-Provincial-Territorial Committee on Drinking Water is reaffirming a MAC of 0.10 mg/L (100 µg/L) for 2,4-D in drinking water based on the following considerations.
- Although an HBV of 0.16 mg/L (160 µg/L) can be calculated, Canadian jurisdictions have shown that a lower MAC of 0.1 mg/L (100 µg/L) is already being consistently achieved.
- No appreciable health benefits would be expected by increasing the MAC;
- Increasing the MAC would provide no reduction in implementation cost and no increase in health protection.
- Analytical methods are available to accurately measure 2,4-D at concentrations well below the MAC.
- Treatment is available to reduce 2,4-D concentrations to the MAC.
The MAC is protective of potential health effects, can be reliably measured by available analytical methods and is achievable by municipal and residential scale treatment technologies. As part of its ongoing guideline review process, Health Canada will continue to monitor new research in this area, including the outcomes of PMRA evaluations, and recommend any change to this guideline technical document that it deems necessary.
8.0 References
Alberta Environment and Sustainable Resource Development (2013). Personal communication with D. LeClair and G. Byrtus.
Alfano, O.M., Brandi, R.J. and Cassano, A.E. (2001). Degradation kinetics of 2,4-D in water employing hydrogen peroxide and UV radiation. Chem. Eng. J., 82(1-3): 209-218.
Amer, S.M. and Aly, F.A.E. (2001). Genotoxic effect of 2,4-dichlorophenoxy acetic acid and its metabolite 2,4-dichlorophenol in mouse. Mutat. Res. Genet. Toxicol. Environ. Mutagen., 494(1-2): 1-12.
APHA, AWWA and WEF (2005). Standard methods for the examination of water and wastewater. 21st edition. Eaton, A.D., Clesceri, L.S., Rice, E.W. and Greenberg, A.E. (eds.). American Public Health Association, American Water Works Association, Water Environment Federation, Washington, DC.
APHA, AWWA and WEF (2012). Standard methods for the examination of water and wastewater. 22nd edition. Rice, E.W., Baird, R.B., Eaton, A.D. and Clesceri, L.S. (eds.). American Public Health Association, American Water Works Association, Water Environment Federation, Washington, DC.
APHA, AWWA and WEF (2017). Standard methods for the examination of water and wastewater. 23rd edition. Baird, R.B., Eaton, A.D. and Rice, E.W. (eds.). American Public Health Association, American Water Works Association, Water Environment Federation, Washington, DC.
APVMA (2006). The reconsideration of approvals and registrations relating to 2,4-D. Australian Government. Canberra, Australia. Available at: https://apvma.gov.au/sites/default/files/publication/14236-2-4-d-phase-7-prf-summary.pdf
Arbuckle, T.E. and Ritter, L. (2005). Phenoxyacetic acid herbicide exposure for women on Ontario farms. J. Toxicol. Environ. Health, Part A, 68(15): 1359-1370.
Arbuckle, T.E., Savitz, D.A., Mery, L.S. and Curtis, K.M. (1999a). Exposure to phenoxy herbicides and the risk of spontaneous abortion. Epidemiology, 10(6): 752-760.
Arbuckle, T.E., Schrader, S.M., Cole, D., Hall, J.C., Bancej, C.M., Turner, L.A. and Claman, P. (1999b). 2,4-Dichlorophenoxyacetic acid residues in semen of Ontario farmers. Repro. Toxicol., 13(6): 421-429.
Arbuckle, T.E., Cole, D.C., Ritter, L. and Ripley, B.D. (2004). Farm children's exposure to herbicides: Comparison of biomonitoring and questionnaire data. Epidemiology, 15(2): 187-194.
Arbuckle, T.E., Cole, D.C., Ritter, L. and Ripley, B.D. (2005). Biomonitoring of herbicides in Ontario farm applicators. Scand. J. Work Environ. Health, 31(SUPPL. 1): 90-97.
Arbuckle, T.E., Bruce, D., Ritter, L. and Hall, C. (2006). Indirect sources of herbicide exposure of families on Ontario farms. J. Expo. Sci. Envion. Epidemiol., 16: 98-104.
Argese, E., Bettiol, C., Marchetto, D., De Vettori, S., Zambon, A., Miana, P. and Ghetti, P.F. (2005). Study on the toxicity of phenolic and phenoxy herbicides using the submitochondrial particle assay. Toxicol. in Vitro, 19(8): 1035-1043.
ASTM (2011). Standard test method for determination of chlorinated organic acid compounds in water by gas chromatography with an electron capture detector. ASTM International, West Conshohocken, Pennsylvania.
Bellamy, W.D., Silverman, G.P., Hendricks, D.W. and Logsdon, G.S. (1985a). Removing Giardia cysts with slow sand filtration. J. Am. Water Works Assoc., 77(2): 52-60.
Bellamy, W.D., Hendricks, D.W. and Logsdon, G.S. (1985b). Slow sand filtration: Influences of selected process variables. J. Am. Water Works Assoc., 77(12): 62-66.
Benitez, F.J., Acero, J.L., Real, F.J. and Roman, S. (2004). Oxidation of MCPA and 2,4-D by UV radiation, ozone, and the combinations UV/H2O2 and O3/H2O2. J. Environ. Sci. Health B, 39(3): 393-409.
Benner, J., Helbling, D.E., Kohler, H-P.E., Wittebol, J., Kaiser, E., Prasse, C., Ternes, T.A., Albers, C.N., Aamand, J., Horemans, B., Springael, D., Walravens, E. and Boon, N. (2013). Is biological treatment a viable alternative for micropollutant removal in drinking water treatment processes? Water Res., 47(16): 5955-5976.
Bharadwaj, L., Dhami, K., Schneberger, D., Stevens, M., Renaud, C. and Ali, A. (2005). Altered gene expression in human hepatoma HepG2 cells exposed to low-level 2,4-dichlorophenoxyacetic acid and potassium nitrate. Toxicol. in Vitro, 19(5): 603-619.
Bloemen, L.J., Mandel, J.S., Bond, G.G., Pollock, A.F., Vitek, R.P. and Cook, R.R. (1993). An update of mortality among chemical workers potentially exposed to the herbicide 2,4-dichlorophenoxyacetic acid and its derivatives. J. Occup. Med., 35(12): 1208-1212.
Bongiovanni, B., Ferri, A., Brusco, A., Rassetto, M., Lopez, L.M., Evangelista de Duffard, A.M. and Duffard, R. (2011). Adverse effects of 2,4-dichlorophenoxyacetic acid on rat cerebellar granule cell cultures were attenuated by amphetamine. Neurotoxic. Res., 19(4): 544-555.
Bortolozzi, A.A., Duffard, R.O., and De Duffard, A.M.E. (1999). Behavioral alteration induced in rats by pre- and postnatal exposure to 2,4-dichlorophenoxyacetic acid. Neurotoxicol. Teratol., 24(4): 451-465.
Bortolozzi, A.A., Duffard, R.O., and De Duffard, A.M.E. (2003). Asymmetrical development of the monoamine system in 2,4-dichlorophenoxyacetic acid treated rats. Neurotoxicology, 24: 149-157.
Bradberry, S.M., Watt, B.E., Proudfoot, A.T. and Vale, J.A. (2000). Mechanisms of toxicity, clinical features, and management of acute chlorophenoxy herbicide poisoning: A review. J. Toxicol. Clin. Toxicol., 38(2): 111-122.
Brahmi, N., Ben Mokhtar, H., Thabet, H., Bouselmi, K. and Amamou, M. (2003). 2,4-D (chlorophenoxy) herbicide poisoning. Vet. Hum. Toxicol., 45(6): 321-322.
Bukowska, B., Rychlik, B., Krokosz, A. and Michalowicz, J. (2008). Phenoxyherbicides induce production of free radicals in human erythrocytes: Oxidation of dichlorodihydrofluorescine and dihydrorhodamine 123 by 2,4-D-Na and MCPA-Na. Food Chem. Toxicol., 46(1): 359-367.
Burns, C.J. and Swaen, G.M. (2012). Review of 2,4-dichlorophenoxyacetic acid (2,4-D) biomonitoring and epidemiology. Crit. Rev. Toxicol., 42(9): 768-786.
Burns, C., Bodner, K., Swaen, G., Collins, J., Beard, K. and Lee, M. (2011). Cancer incidence of 2,4-D production workers. Int. J. Environ. Res. Public Health, 8(9): 3579-3590.
Cantor, K.P., Blair, A., Everett, G., Gibson, R., Burmeister, L.F., Brown, L.M., Schuman, L. and Dick, F.R. (1992). Pesticides and other agricultural risk factors for non-Hodgkin's lymphoma among men in Iowa and Minnesota. Cancer Res., 52(9): 2447-2455.
Carreon, R.E., Johnson, K.A. and Wall, J.M. (1983). 2,4-Dichlorophenoxy-acetic acid isopropylamine salt: Acute toxicological properties. Unpublished report from The Dow Chemical Company, Midland, MI, USA. Submitted to WHO by Industry Task Force II on 2,4-D Research Data, Indianapolis, IN, USA (as cited in: JMPR, 1996).
CCME (2004). From source to tap: Guidance on the multi-barrier approach to safe drinking water. Canadian Council of Ministers of the Environment, Winnipeg, Manitoba.
Chamberlain, E., Shi, H., Wang, T., Ma, Y., Fulmer, A. and Adams, C. (2012). Comprehensive screening study of pesticide degradation via oxidation and hydrolysis. J. Agric. Food Chem., 60(1): 354-363.
Charles, J.M., Bond, D.M., Jeffries, T.K., Yano, B.L., Stott, W.T., Johnson, K.A., Cunny, H.C., Wilson, R.D. and Bus, J.S. (1996a). Chronic dietary toxicity/oncogenicity studies on 2,4-dichlorophenoxyacetic acid in rodents. Fundam. Appl. Toxicol., 33(2): 166-172.
Charles, J., Cunny, H., Wilson, R. and Bus, J.S. (1996b). Comparative subchronic studies on 2,4-dichlorophenoxyacetic acid, amine and ester in rats. Fundam. Appl. Toxicol., 33(2): 161-165.
Charles, J.M., Cunny, H.C., Wilson, R.D., Bus, J.S., Lawlor, T.E., Cifone, M.A., Fellows, M. and Gollapudi, B. (1999a). Ames assays and unscheduled DNA synthesis assays on 2,4-dichlorophenoxyacetic acid and its derivatives. Mutat. Res. Genet. Toxicol. Environ. Mutagen., 444(1): 207-216.
Charles, J.M., Cunny, H.C., Wilson, R.D., Ivett, J.L., Murli, H., Bus, J.S. and Gollapudi, B. (1999b). In vivo micronucleus assays on 2,4-dichlorophenoxyacetic acid and its derivatives. Mutat. Res. Genet. Toxicol. Environ. Mutagen., 444(1): 227-234.
Charles, J.M., Hanley Jr., T.R., Wilson, R.D., van Ravenzwaay, B. and Bus, J.S. (2001). Developmental toxicity studies in rats and rabbits on 2,4-dichlorophenoxyacetic acid and its forms. Toxicol. Sci., 60(1): 121-131.
Chowdhury, Z., Traviglia, A., Carter, J., Brown, T., Summers, R.S., Corwin, C.J., Zearley, T., Thurman, M., Ferrara, I., Olson, J., Thacker, R. and Barron, P. (2010). Cost-effective regulatory compliance with GAC biofilters. Report No. 4155. Water Research Foundation, Denver, Colorado.
Chowdhury, Z.K., Summers, R.S., Westerhoff, G.P., Leto, B.J., Nowack, K.O. and Corwin, C.J. (2013). Activated carbon: Solutions for improving water quality. Passantino, L. B. (ed.). American Water Works Association. Denver, Colorado.
Clausen, L. (2000). Retention of pesticides in filter membranes. J. Environ. Qual., 29(2): 654-657.
Clausen, M., Leier, G. and Witte, I. (1990). Comparison of the cytotoxicity and DNA-damaging properties of 2,4-D and U 46 D Fluid (dimethylammonium salt of 2,4-D). Arch. Toxicol., 64(6): 497-501.
Coady, K.K., Kan, H.L., Schisler, M.R., Gollapudi, B.B., Neal, B., Williams, A. and LeBaron, M.J. (2014). Evaluation of potential endocrine activity of 2,4-dichlorophenoxyacetic acid using in vitro assays. Toxicol. in Vitro, 28(5): 1018-1025.
Corwin, C.J. and Summers, R.S. (2012). Controlling trace organic contaminants with GAC adsorption. J. Am. Water Works Assoc., 104(1): E36-E47.
Croll, B.T., Chadwick, B. and Knight, B. (1992). The removal of atrazine and other herbicides from water using granular activated carbon. Water Sci. Technol. Water Supply 10(2): 111-120.
Dalgard, D.W. (1993). 52 Week dietary toxicity study with 2,4-D acid in dogs. Unpublished report No. 2184-124 from Hazleton Laboratories America, Inc., Vienna, VA, USA. Submitted to WHO by Industry Task Force II on 2,4-D Research Data, Indianapolis, IN, USA (as cited in JMPR, 1996).
De Duffard, A.M., Brusco, A., Duffard, R., Garcia, G., and Pecci Saavedra, J. (1995). Changes in serotonin-immunoreactivity in the dorsal and median raphe nuclei of rats exposed to 2,4-Dichlorophenoxy acetic acid through lactation. Mol. Chem. Neuropathol., 26: 187-193.
De Roos, A.J., Zahm, S.H., Cantor, K.P., Weisenburger, D.D., Holmes, F.F., Burmeister, L.F. and Blair, A. (2003). Integrative assessment of multiple pesticides as risk factors for non-Hodgkin's lymphoma among men. J. Occup. Environ. Med., 60(9): e11.
Dhillon, A.S., Tarbutton, G.L., Levin, J.L., Plotkin, G.M., Lowry, L.K., Nalbone, J.T. and Shepherd, S. (2008). Pesticide/environmental exposures and Parkinson's disease in East Texas. J. Agromedicine, 13(1): 37-48.
Donald, D.B., Cessna, A.J., Sverko, E. and Glozier, N.E. (2007). Pesticides in surface drinking-water supplies of the Northern Great Plains. Environ. Health Perspect., 115: 1183-1191.
Duchnowicz, P. and Koter, M. (2003). Damage to the erythrocyte membrane caused by chlorophenoxyacetic herbicides. Cell. Mol. Biol. Lett., 8(1): 25-30.
Duffard, R., Rosso, G.S., Bortolozzy, A., Madariaga, M., Di Paolo, O., and De Duffard, A.M.E. (1996). Central nervous system myelin deficit in rats exposed to 2,4-dichlorophenoxy acetic acid throughout lactation. Neurotoxicol. Teratol., 18(6): 691-696.
Durakovic, Z., Durakovic, A., Durakovic, S. and Ivanovic, D. (1992). Poisoning with 2,4-dichlorophenoxyacetic acid treated by hemodialysis. Arch. Toxicol., 66(7): 518-521.
Edwards, V.H. and Schubert, P.F. (1974). Removal of 2,4-D and other persistent organic molecules from water supplies by reverse osmosis. J. Am. Water Works Assoc., 66(10): 610-616.
Environment Canada (2011). Presence and levels of priority pesticides in selected Canadian aquatic ecosystems. Water Science and Technology Directorate. Cat. No.: En14-40/2011E-PDF.
Eriksson, M., Hardell, L., Carlberg, M. and Åkerman, M. (2008). Pesticide exposure as risk factor for non-Hodgkin lymphoma including histopathological subgroup analysis. Int. J. Cancer, 123(7): 1657-1663.
Erne, K. (1966). Distribution and elimination of chlorinated phenoxyacetic acids in animals. Acta Vet. Scand., 7: 240-256.
European Union (2020). Council Directive 2020/2184 of the European Parliament and of the Council of 16 December 2020 on the quality of water intended for human consumption (recast).
Feldmann, R.J. and Maibach, H.I. (1974). Percutaneous penetration of some pesticides and herbicides in man. Toxicol. Appl. Pharmacol., 28(1): 126-132.
Ferri, A., Duffard, R. and de Duffard, A.M.E. (2007). Selective oxidative stress in brain areas of neonate rats. Drug Chem. Toxicol., 30(1): 17-30.
Flanagan, R.J., Meredith, T.J., Ruprah, M., Onyon, L.J. and Liddle, A. (1990). Alkaline diuresis for acute poisoning with chlorophenoxy herbicides and ioxynil. Lancet, 335(8687): 454-458.
Foster, D.M., Rachwal, A.J. and White, S.L. (1991). New treatment processes for pesticides and chlorinated organics control in drinking water. J. Inst. Water Environ. Manage., 5(4): 466-476.
Foster, D.M., Rachwal, A.J. and White, S.L. (1992). Advanced treatment for the removal of atrazine and other pesticides. Water Sci. Technol. Water Supply, 10(2): 133-146.
Frantz, S.W. and Kropscott, B.E. (1993). Pharmacokinetic evaluation of a single oral administration of the 2-ethylhexyl (isooctyl) ester of 2,4-D to Fischer 344 rats. J. Occup. Med. Toxicol., 2(1): 75-85.
Frick, E. A. and Dalton, M.S. (2005). Characterization of anthropogenic organic compounds in the source water and finished water for the City of Atlanta, October 2002-September 2004. In: Hatcher, K.S. (ed.) Proceedings of the 2005 Georgia Water Resources Conference, April 25-27, Institute of Ecology, University of Georgia, Athens, Georgia. Available at: https://smartech.gatech.edu/bitstream/handle/1853/47123/FrickE%20paper%20March15%20rev.pdf
Friesen, E.G., Jones, G.R. and Vaughan, D. (1990). Clinical presentation and management of acute 2,4-D oral ingestion. Drug Saf., 5(2): 155-159.
Garry, V.F., Schreinemachers, D., Harkins, M.E. and Griffith, J. (1996). Pesticide appliers, biocides, and birth defects in rural Minnesota. Environ. Health Perspect., 104(4): 394-399.
Giri, R.R., Ozaki, H., Ishida, T., Takanami, R. and Taniguchi, S. (2007). Synergy of ozonation and photocatalysis to mineralize low concentration 2,4-dichlorophenoxyacetic acid in aqueous solution. Chemosphere, 66(9): 1610-1617.
Giroux, I. (2002). Contamination de l'eau par les pesticides dans les régions de culture de maïs et de soya au Québec, Résultats des campagnes d'échantillonnage de 1999, 2000 et 2001 et évolution temporelle de 1992 à 2001. Gouvernement de Québec, Ministère de l'Environnement. ENV/2002/0365, QE/137, ISBN 2-550-40286-3.
Giroux, I. and Therrien, M. (2005). Les pesticides utilisés dans les espaces verts urbains: présence dans l'eau des rejets urbains et dans l'air ambiant. Direction du suivi de l'état de l'environnement, Ministère du Développement durable, de l'Environnement et des Parcs, ENV/2005/0165. QE/164.
Glozier, N.E., Struger, J., Cessna, A.J., Gledhill, M., Rondeau, M., Ernst, W.R., Sekela, M.A., Cagampan, S.J., Sverko, E., Murphy, C., Murray, J.L. and Donald, D.B. (2012). Occurrence of glyphosate and acidic herbicides in select urban rivers and streams in Canada, 2007. Environ. Sci. Pollut. Res. Int., 19(3): 821-834.
Gollapudi, B.B., Charles, J.M., Linscombe, V.A., Day, S.J. and Bus, J.S. (1999). Evaluation of the genotoxicity of 2,4-dichlorophenoxyacetic acid and its derivatives in mammalian cell cultures. Mutat. Res. Genet. Toxicol. Environ. Mutagen., 444(1): 217-225.
Gonzalez, M., Soloneski, S., Reigosa, M.A. and Larramendy, M.L. (2005). Genotoxicity of the herbicide 2,4-dichlorophenoxyacetic and a commercial formulation, 2,4-dichlorophenoxyacetic acid dimethylamine salt. I. Evaluation of DNA damage and cytogenetic endpoints in Chinese Hamster ovary (CHO) cells. Toxicol. in Vitro, 19(2): 289-297.
Goodman, J.E., Loftus, C.T. and Zu, K. (2015). 2,4-Dichlorophenoxyacetic acid and non-Hodgkin's lymphoma, gastric cancer, and prostate cancer: Meta-analyses of the published literature. Ann. Epidemiol., 25(8): 626-636.
Goodman, J.E., Loftus, C.T. and Zu, K. (2017). 2,4-Dichlorophenoxyacetic acid and non-Hodgkin's lymphoma: results from the Agricultural Health Study and updated meta-analyses. Ann. Epidemiol., 27(4): 626-636.
Gorzinski, S.J., Kociba, R.J., Campbell, R.A., Smith, F.A., Nolan, R.J. and Eisenbrandt, D.L. (1987). Acute, pharmacokinetic, and subchronic toxicological studies of 2,4-dichlorophenoxyacetic acid. Fundam. Appl. Toxicol., 9(3): 423-435.
Griffin, R.J., Godfrey, V.B., Kim, Y.C. and Burka, L.T. (1997). Sex-dependent differences in the disposition of 2,4-dichlorophenoxyacetic acid in Sprague-Dawley rats, B6C3F1 mice, and Syrian hamsters. Drug Metab. Dispos., 25(9): 1065-1071.
Greene, B.E., Snoeyink, V.L. and Pogge, F.W. (1994). Adsorption of pesticides by powdered activated carbon. American Water Works Association Research Foundation and American Water Works Association, Denver, Colorado.
Gustafson, D.K., Carr, K.H., Carson, D.B., Fuhrman, J.D., Hackett, A.G., Hoogheem, T.J., Snoeyink, V.L., Curry, M., Heijman, B., Chen, S., Herti, P. and van Wesenbeeck, I. (2003). Activated carbon adsorption of chloroacetanilide herbicides and their degradation products from surface water supplies. J. Water Supply Res. Technol. AQUA, 52(6): 443-454.
Haist-Gulde, B. (2014). Personal communication, Technologiezentrum Wasser, Karlsruher, Germany.
Haist-Gulde, B. and Happel, O. (2012). Removal of pesticides and their ionic degradates by adsorptive processes. Report no. 4022. Water Research Foundation, Denver, Colorado.
Haist-Gulde, B., Baldauf, G. and Brauch, H.-J. (1993). Removal of pesticides from raw waters. Water Sci. Technol. Water Supply, 11(1): 187-196.
Hancock, D.B., Martin, E.R., Mayhew, G.M., Stajich, J.M., Jewett, R., Stacy, M.A., Scott, B.L., Vance, J.M. and Scott, W.K. (2008). Pesticide exposure and risk of Parkinson's disease: A family-based case-control study. BMC Neurol., 8: 6.
Hardell, L. and Sandstrom, A. (1979). Case-control study: soft-tissue sarcomas and exposure to phenoxyacetic acids or chlorophenols. Br. J. Cancer, 39(6): 711-717.
Hardell, L. (1981). Relation of soft-tissue sarcoma, malignant lymphoma and colon cancer to phenoxy acids, chlorophenols and other agents. Scand. J. Work Environ. Health, 7(2): 119-130.
Hardell, L. and Eriksson, M. (1999). A case-control study of non-Hodgkin lymphoma and exposure to pesticides. Cancer, 85(6): 1353-1360.
Harris, S.A. and Solomon, K.R. (1992). Percutaneous penetration of 2,4-dichlorophenoxyacetic acid and 2,4-D dimethyamine salt in human volunteers. J. Toxicol. Environ. Health, 36: 233-240.
Hasegawa, M., Kusuhara, H., Endou, H. and Sugiyama, Y. (2003). Contribution of organic anion transporters to the renal uptake of anionic compounds and nucleoside derivatives in rat. J. Pharmacol. Exp. Ther., 305(3): 1087-1097.
HSDB, Hazardous Substances Databank (2005). 2,4-D, dimethylamine. CASRN: 2008-39-1. US National Library of Medicine, Division of Specialized Information Services, Bethesda, MD. Available at: https://toxnet.nlm.nih.gov/cgi-bin/sis/search/a?dbs+hsdb:@term+@DOCNO+2599
HSDB, Hazardous Substances Databank (2015). 2,4-D. CASRN: 94-75-7. US National Library of Medicine, Division of Specialized Information Services, Bethesda, MD. Available at: http://toxnet.nlm.nih.gov/cgi-bin/sis/search2/r?dbs+hsdb:@term+@DOCNO+202
Health Canada (1994). Canadian Environmental Protection Act. Human health risk assessment for priority substances. Minister of Supply and Services Canada, Ottawa, Ontario.
Health Canada (2005a). Proposed acceptability for continuing registration: Re-evaluation of the lawn and turf uses of (2,4-dichlorophenoxy)acetic acid [2,4-D]. PACR2005-01. Pest Management Regulatory Agency (PMRA), Health Canada, Ottawa, Ontario.
Health Canada (2005b). Memorandum: Toxicology re-evaluation of additional data submitted by the 2,4-D TF II in response to issues raised by the PMRA during the 2,4-D turf assessment (PACR2005-01). Internal communication.
Health Canada (2006). Re-evaluation Decision. RVD2006-11. Lawn and turf uses of (2,4-Dichlorophenoxy)acetic acid [2,4-D]. Interim measures. Pest Management Regulatory Agency (PMRA); Health Canada, Ottawa, Ontario. Available at: http://publications.gc.ca/collections/Collection/H113-5-2006-11E.pdf
Health Canada (2007). Proposed acceptability for continuing registration: Re-evaluation of the agricultural, forestry, aquatic and industrial site uses of (2,4-dichlorophenoxy)acetic acid [2,4-D]. PACR2007-06. Pest Management Regulatory Agency (PMRA), Health Canada, Ottawa, Ontario.
Health Canada (2008). Re-evaluation Decision. RVD2008-11. 2,4-Dichlorophenoxy)acetic acid [2,4-D]. Pest Management Regulatory Agency (PMRA); Health Canada, Ottawa, Ontario. Available at: https://24d.info/wp-content/uploads/2020/08/2008-PMRA-Re-evaluation-Decision.pdf
Health Canada (2013). Second report on human biomonitoring of environmental chemicals in Canada. Results of the Canadian Health Measures Survey Cycle 2 (2009-2011). 978-1-100-22140-3. Available at: http://publications.gc.ca/site/eng/442162/publication.html
Health Canada (2016). Re-evaluation Note. REV2016-08, Special review of 2,4-D: Proposed decision for consultation. PMRA, Health Canada, Ottawa, Ontario., ISSN: 1925-0649. Catalogue number: H113-5/2016-8E-PDF. Available at: www.canada.ca/en/health-canada/services/consumer-product-safety/pesticides-pest-management/public/consultations/re-evaluation-note/2016/special-review-2-4-d/document.html
Health Canada (2018a). Residue limits for pesticides database: 2,4-D. Health Canada, Consumer Product Safety, Pesticides and Pest Management. Available at: http://pr-rp.hc-sc.gc.ca/mrl-lrm/index-eng.php
Health Canada (2018b). Personal communication from the Health Evaluation Directorate, Pest Management Regulatory Agency (PMRA).
Health Canada (2018c). Personal communication from the Health Evaluation Directorate, Pest Management Regulatory Agency (PMRA).
Health Canada (2019). Personal communication from the Health Evaluation Directorate, Pest Management Regulatory Agency (PMRA).
Health Canada (2020). Pest control products sales report for 2018. PMRA, Health Canada, Ottawa, Ontario.
Heath Canada (2021). Canadian exposure factors used in human health risk assessments. Fact Sheet. Health Canada, Ottawa, Ontario. Available at: https://www.canada.ca/en/health-canada/services/chemical-substances/fact-sheets/canadian-exposure-factors-human-health-risk-assessments.html
Hoar, S.K., Blair, A., Holmes, F.F., Boysen, C.D., Robel, R.J., Hoover, R. and Fraumeni, J.F. (1986). Agricultural herbicide use and risk of lymphoma and soft-tissue sarcoma. J. Am. Med. Assoc., 256(9): 1141-1147.
Hu, J., Morita, T., Magara, Y. and Aizawa, T. (2000). Evaluation of reactivity of pesticides with ozone in water using the energies of frontier molecular orbitals. Wat. Res., 34(8): 2215-2222.
Hughes, W.B. and Younker, C.L. (2011). Organic compounds assessed in Chattahoochee River water used for public supply near Atlanta, Georgia, 2004-05. Fact Sheet 2011-3062. US Geological Survey, Reston, Virginia. 6p.
Huntscha, S., Rodriguez Velosa, D.M., Schroth, M.H. and Hollender, J. (2013). Degradation of polar organic micropollutants during riverbank filtration: Complementary results from spatiotemporal sampling and push-pull tests. Environ. Sci. Technol., 47(20): 11512-11521.
IARC, International Agency for Research on Cancer (2017). IARC monographs on the evaluation of carcinogenic risks to humans: DDT, lindane, and 2,4-D. Volume 113. IARC Working Group on the Evaluation of Carcinogenic Risks to Humans (ed.). IARC Press, Lyons, France, p. 480.
Jeffries, T.K., Yano, B.L., Ormand, J.R. and Battjes, J.E. (1995). 2,4-D: Chronic toxicity/oncogenicity study in Fischer 344 rats (Unpublished study). The Dow Chemical Company, Health and Environmental Sciences. Midland, MI (as cited in JMPR, 1996 and US EPA, 1996).
JMPR (1996). Pesticides residues in food: 1996 evaluations Part II Toxicology. International Programme on Chemical Safety-INCHEM (IPCS) Rowland, J.C. (ed.). US Environmental Protection Agency, Washington, DC. Available at: http://www.inchem.org/documents/jmpr/jmpmono/v96pr04.htm
Johnson, W.G., Lavy, T.L. and Gbur, E.E. (1995). Sorption, mobility and degradation of trichlopyr and 2,4-D on four soils. Weed Science, 43(4): 678-684.
Kamel, F., Tanner, C., Umbach, D., Hoppin, J., Alavanja, M., Blair, A., Comyns, K., Goldman, S., Korell, M., Langston, J., Ross, G. and Sandler, D. (2007). Pesticide exposure and self-reported Parkinson's disease in the Agricultural Health Study. Am. J. Epidemiol., 165(4): 364-374.
Kancir, C.B., Andersen, C. and Olesen, A.S. (1988). Marked hypocalcemia in a fatal poisoning with chlorinated phenoxy acid derivatives. J. Toxicol. Clin. Toxicol., 26(3-4): 257-264.
Kaya, B., Yanikoglu, A. and Marcos, R. (1999). Genotoxicity studies on the phenoxyacetates 2,4-D and 4-CPA in the Drosophila wing spot test. Teratog. Carcinog. Mutagen., 19(4): 305-312.
Keller, T., Skopp, G., Wu, M. and Aderjan, R. (1994). Fatal overdose of 2,4-dichlorophenoxyacetic acid (2,4-D). Forensic Sci. Int., 65(1): 13-18.
Kennedy, A., Reinert, A., Summers, R.S., Ferrer, I., Thurman, M. and Knappe, D.R.U. (2012). Scale-up of the GAC adsorption of trace organic compounds. Proceedings of the 2012 WQTC Conference, Toronto, Ontario. American Water Works Association, Denver, Colorado.
Kennepohl, E., Munro, I.C. and Bus, J.S. (2010). Chapter 84: phenoxy herbicides (2,4-D). In: Hayes' handbook of pesticide toxicology. 3rd edition. Krieger, R. (ed.). Academic Press, New York, pp. 1829-1847.
Knappe, D.R.U., Snoeyink, V.L., Roche, P., Prados, M.J. and Bourbigot, M-M. (1999). Atrazine removal by preloaded GAC. J. Am. Water Works Assoc., 91(10): 97-109.
Kogevinas, M., Becher, H., Benn, T., Bertazzi, P.A., Boffetta, P., Bueno-de-Mesquita, H.B., Coggon, D., Colin, D., Flesch-Janys, D., Fingerhut, M., Green, L., Kauppinen, T., Littorin, M., Lynge, E., Mathews, J.D., Neuberger, M., Pearce, N. and Saracci, R. (1997). Cancer mortality in workers exposed to phenoxy herbicides, chlorophenols, and dioxins. An expanded and updated international cohort study. Am. J. Epidemiol., 145(12): 1061-1075.
Kohli, J.D., Khanna, R.N., Gupta, B.N., Dhar, M.M., Tandon, J.S. and Sircar, K.P. (1974). Absorption and excretion of 2,4-dichlorophenoxyacetic acid in man. Xenobiotica, 4(2): 97-100.
Kouras, A., Zouboulis, A., Samara, C. and Kouimtzis, Th. (1998). Removal of pesticides from aqueous solutions by combined physiochemical processes-the behaviour of lindane. Environ. Pollut., 103(2-3): 193-202.
Krishnan, K. and Carrier, R. (2013). The use of exposure source allocation factor in the risk assessment of drinking-water contaminants. J. Toxicol. Environ. Health B, 16(1): 39-51.
Kruithof, J.C., Kamp, P.C. and Belosevic, M. (2002). UV/H2O2-treatment: the ultimate solution for pesticide control and disinfection. J. Water Supply Res. Technol. AQUA, 2(1): 113-122.
Kumar, Y. (2001). Pesticides in ambient air in Alberta. Report prepared for the Air Research Users Group, Alberta Environment, Edmonton, Alberta. ISBN 0-7785-1889-4.
Lee, K., Johnson, V.L. and Blakley, B.R. (2001). The effect of exposure to a commercial 2,4-D formulation during gestation on the immune response in CD-1 mice. Toxicology, 165(1): 39-49.
Lerda, D. and Rizzi, R. (1991). Study of reproductive function in persons occupationally exposed to 2,4-dichlorophenoxyacetic acid (2,4-D). Mutat. Res., 262: 47-50.
Linnainmaa, K. (1984). Induction of sister chromatid exchanges by the peroxisome proliferators 2,4-D, MCPA, and clofibrate in vivo and in vitro. Carcinogenesis, 5(6): 703-707.
Logsdon, G.S., Kohne, R., Abel, S. and LaBonde, S. (2002). Slow sand filtration for small water systems. J. Environ. Eng. Sci., 1(5): 339-348.
Loomis, D., Guyton, K., Grosse, Y., El Ghissasi, F., Bouvard, V., Benbrahim-Tallaa, L., Guha, N., Mattock, H. and Straif, K. (2015). Carcinogenicity of lindane, DDT, and 2,4-dichlorophenoxyacetic acid. Lancet Oncol., 16(8): 891-892.
Manitoba Conservation and Water Stewardship (2013). Personal communication with K. Philip and N. Armstrong.
Marouani, N., Tebourbi, O., Cherif, D., Hallegue, D., Yacoubi, M.T., Sakly, M., Benkhalifa, M. and Ben Rhouma, K. (2017). Effects of oral administration of 2,4-dichlorophenoxyacetic acid (2,4-D) on reproductive parameters in male Wistar rats. Environ. Sci. Pollut. Res. Int., 24(1): 519-526.
Marty, M.S., Neal, B.H., Zablotny, C.L., Yano, B.L., Andrus, A.K., Woolhiser, M.R., Boverhof, D.R., Saghir, S.A., Perala, A.W., Passage, J.K., Lawson, M.A., Bus, J.S., Lamb, J.C. 4th and Hammond, L. (2013). An F1-extended one-generation reproductive toxicity study in Crl:CD(SD) rats with 2,4-dichlorophenoxyacetic acid. Toxicol. Sci., 136(2): 527-547.
Mattsson, J.L., Charles, J.M., Yano, B.L., Cunny, H.C., Wilson, R.D. and Bus, J.S. (1997). Single-dose and chronic dietary neurotoxicity screening studies on 2,4-dichlorophenoxyacetic acid in rats. Fundam. Appl. Toxicol., 40(1): 111-119.
Meijers, R.T., Oderwald-Muller, E.J., Nuhn, P.A.N.M. and Kruithof, J.C. (1995). Degradation of pesticides by ozonation and advanced oxidation. Ozone Sci. Eng., 17(6): 673-686.
Miligi, L., Costantini, A.S., Bolejack, V., Veraldi, A., Benvenuti, A., Nanni, O., Ramazzotti, V., Tumino, R., Stagnaro, E., Rodella, S., Fontana, A., Vindigni, C. and Vineis, P. (2003). Non-Hodgkin's lymphoma, leukemia, and exposures in agriculture: Results from the Italian multicenter case-control study. Am. J. Ind. Med., 44(6): 627-636.
Miligi, L., Costantini, A.S., Veraldi, A., Benvenuti, A., Vineis, P., Nanni, O., Ramazzotti, V., Tumino, R., Stagnaro, E., Rodella, S., Fontana, A. and Vindigni, C. (2006). Cancer and pesticides: An overview and some results of the Italian multicenter case-control study on hematolymphopoietic malignancies. Ann. NY Acad. Sci., 1076: 366-377.
Mills, P.K. and Yang, R.C. (2007). Agricultural exposures and gastric cancer risk in Hispanic farm workers in California. Environ. Res., 104(2): 282-289.
Mills, P.K., Yang, R. and Riordan, D. (2005). Lymphohematopoietic cancers in the United Farm Workers of America (UFW), 1988-2001. Cancer Causes Control, 16(7): 823-830.
Miltner, R.J., Baker, D.B., Speth, T.F. and Fronk, C.A. (1989). Treatment of seasonal pesticides in surface waters. J. Am. Water Works Assoc., 81(1): 43-52.
Ministère de l'Environnement et de la Lutte contre les Changements climatiques (2021). Personal communication with P. Cantin.
Moody, R., Franklin, C., Ritter, L. and Maibach, H. (1990). Dermal absorption of the phenoxy herbicides 2,4-D, 2,4-D amine, 2,4-D isooctyl and 2,4,5-T in rabbits, rats, rhesus monkeys and humans: A cross-species comparison. J. Toxicol. Environ. Health, 29: 237-245.
Moody, R.P., Wester, R.C., Melendres, J.L. and Maibach, H.I. (1992). Dermal absorption of the phenoxy herbicide 2,4-D dimethylamine in humans: Effect of DEET and anatomic site. J. Toxicol. Environ. Health, 36: 241-250.
Morrison, H.I., Wilkins, K., Semenciw, R., Mao, Y. and Wigle, D. (1992). Herbicides and cancer. J. Natl. Cancer Inst., 84: 1866-1874.
Munro, I.C., Carlo, G.L., Orr, J.C., Sund, K.G., Wilson, R.M., Kennepohl, E., Lynch, B.S, Jablinske, M.and Lee, N.L. (1992). A comprehensive, integrated review and evaluation of the scientific evidence relating to the safety of the herbicide 2,4-D. J. Am. Coll. Toxicol., 11(5): 559-664.
Mustonen, R., Kangas, J., Vuojolahti, P. and Linnainmaa, K. (1986). Effects of phenoxyacetic acids on the induction of chromosome aberrations in vitro and in vivo. Mutagenesis, 1(4): 241-245.
Nakbi, A., Tayeb, W., Dabbou, S., Chargui, I., Issaoui, M., Zakhama, A., Miled, A. and Hammami, M. (2012). Hypolipidimic and antioxidant activities of virgin olive oil and its fractions in 2,4-diclorophenoxyacetic acid-treated rats. Nutrition, 28(1): 81-91.
Neal, B.H., Bus, J., Marty, M.S., Coady, K., Williams, A., Staveley, J. and Lamb, J.C. (2017). Weight-of-the-evidence of 2,4-D potential for interactions with the estrogen, androgen and thyroid pathways and steroidogenesis. Crit. Rev. Toxicol., 47(5): 354-401.
Nielsen, K., Kæmpe, B., and Jensen-Holm, J. (1965). Fatal poisoning in man by 2,4-dichlorophenoxyacetic acid (2,4-D): Determination of the agent in forensic materials. Acta Pharmacol. Toxicol., 22: 224-234.
New Brunswick Department of Health (2013). Personal communication with K. Gould.
NHMRC, NRMMC (2011) National Water Quality Management Strategy: Australian Drinking Water Guidelines Paper 6. National Health and Medical Research Council, National Resource Management Ministerial Council, Commonwealth of Australia, Canberra.
Nova Scotia Environment (2021). Personal communication with A. Polegato.
Nozaki, Y., Kusuhara, H., Kondo, T., Hasegawa, M., Shiroyanagi, Y., Nakazawa, H., Okano, T. and Sugiyama, Y. (2007). Characterization of the uptake of organic anion transporter (OAT) 1 and OAT3 substrates by human kidney slices. J. Pharmacol. Exp. Ther., 321(1): 362-369.
NSF/ANSI (2018a). NSF International/American National Standards Institute Standard 53: Drinking water treatments units-Health effects. NSF International, Ann Arbor, Michigan.
NSF/ANSI (2018b). NSF International/American National Standards Institute Standard 58: Reverse osmosis drinking water treatment systems. NSF International, Ann Arbor, Michigan.
Ntzani, E.E., Ntritsos, C.M., Evangelou, G. and Tzoulaki, E. (2013). Literature review on epidemiological studies linking exposure to pesticides and health effects. EFSA Supporting Publication 2013, 10(10): EN-497.
OEHHA (2009). Public health goal for 2,4-dichlorophenoxyacetic acid in drinking water. Pesticide and Environmental Toxicology Branch Office of Environmental Health Hazard Assessment, California Environmental Protection Agency, California.
Ontario Ministry of the Environment (2021). Personal communication with S. Deshpande.
O'Reilly, J.F. (1984). Prolonged coma and delayed peripheral neuropathy after ingestion of phenoxyacetic acid weedkillers. Postgrad. Med. J., 60(699): 76-77.
Pahwa, P., Karunanayake, C.P., Dosman, J.A., Spinelli, J.J. and McLaughlin, J.R. (2011). Soft-tissue sarcoma and pesticides exposure in men: Results of a Canadian case-control study. J. Occup. Environ. Med., 53(11): 1279-1286.
Palmeira, C.M., Moreno, A.J. and Madeira, V.M.C. (1995). Thiols metabolism is altered by the herbicides paraquat, dinoseb and 2,4-D: A study in isolated hepatocytes. Toxicol. Lett., 81(2-3): 115-123.
Pavlica, M., Papes, D. and Nagy, B. (1991). 2,4-Dichlorophenoxyacetic acid causes chromatin and chromosome abnormalities in plant cells and mutation in cultured mammalian cells. Mutat. Res., 263(2): 77-81.
Plakas, K.V. and Karabelas, A. J. (2012). Removal of pesticides from water by NF and RO membranes-A review. Desalination, 287: 255-265.
Pochettino, A.A., Bongiovanni, B., Duffard, R.O. and Evangelista de Duffard, A.M. (2013). Oxidative stress in ventral prostate, ovary, and breast by 2,4-dichlorophenoxyacetic acid in pre- and postnatal exposed rats. Environ. Toxicol., 28(1): 1-10.
Pochettino, A.A., Hapon, M.B., Biolatto, S.M., Madariaga, M.J., Jahn, G.A. and Konjuh, C.N. (2016). Effects of 2,4-dichlorophenoxyacetic acid on the ventral prostate of rats during the peri-pubertal, pubertal and adult stage. Drug Chem. Toxicol., 39(4): 392-399.
Prado, A.G.S, Vieria, E.M., and Olimpia de O. Rezende, M. (2001). Monitoring of the harmful concentrations of 2,4-dichlorophenoxyacetic acid (2,4-D) in soils with and without organic matter. J. Braz. Chem. Soc., 12(4): 485-488.
Qurratu, A. and Reehan, A. (2016). A review of 2,4-dichlorophenoxyacetic acid (2,4-D) derivatives: 2,4-D dimethylamine salt and 2,4-D butyl ester. Int. J. Appl. Engineer. Res., 11(19): 9946-9955.
Robeck, G., Dostal, K.A., Cohen, J.M. and Kreissl, J.F. (1965). Effectiveness of water treatment processes in pesticide removal. J. Am. Water Works Assoc., 57(2): 181-199.
Rodwell, D.E. (1985). A dietary two-generation reproduction study in Fischer 344 rats with dichlorophenoxy acetic acid. Unpublished Report No. WIL-81137 from WIL Research Laboratories, Inc., OH, USA. Submitted to WHO by Industry Task Force II on 2,4-D Research Data, Indianapolis, IN, USA (as cited in: JMPR, 1996).
Rosso, S.B., Di Paolo, O.A., De Duffard, A.M.E., and Duffard, R. (1997). Effects of 2,4-dichlorophenoxy acetic acid on central nervous system of developmental rats. Associated changes in ganglioside pattern. Brain Res., 769: 163-167.
Rosso, S.B., Garcia, G.B., Madariaga, M.J., De Duffard, A.M.E., and Duffard, R.O. (2000). 2,4-Dichlorophenoxy acetic acid in developing rats alters behaviour, myelination and regions brain gangliosides pattern. Neurotoxicology, 21:155-164.
Rugbjerg, K., Harris, M.A., Shen, H., Marion, S.A., Tsui, J.K.C. and Tesche, K. (2011). Pesticide exposure and risk of Parkinson's disease-A population-based case-control study evaluating the potential for recall bias. Scand. J. Work Environ. Health, 37(5): 427-236.
Saghir, S.A., Marty, M.S., Zablotny, C.L., Passage, J.K., Perala, A.W., Neal, B.H., Hammond, L. and Bus, J.S. (2013). Life-stage-, sex-, and dose-dependent dietary toxicokinetics and relationship to toxicity of 2,4-dichlorophenoxyacetic acid (2,4-D) in rats: Implications for toxicity test dose selection, design, and interpretation. Toxicol. Sci., 136(2): 297-307.
Saskatchewan Water Security Agency (2021). Personal communication with A. Thirunavukkarasu.
Sauerhoff, M.W., Braun, W.H., Blau, G.E. and Gehring, P.J. (1977). The fate of 2,4-dichlorophenoxyacetic acid (2,4-D) following oral administration to man. Toxicology, 8(1): 3-11.
SCC (2019). Directory of accredited product, process and service certification bodies. Standards Council of Canada, Ottawa, Ontario. Available at: www.scc.ca/en/accreditation/product-process-and-service-certification/directory-of-accredited-clients
Schinasi, L. and Leon, M.E. (2014). Non-Hodgkin lymphoma and occupational exposure to agricultural pesticide chemical groups and active ingredients: A systematic review and meta-analysis. Int. J. Environ. Res. Public Health, 11(4): 4449-4527.
Schultze, G.E. (1991a) Subchronic toxicity study in mice with 2,4-D acid. Unpublished report No. 2184-117 from Hazleton Laboratories America, Inc., Vienna, VA, USA submitted to WHO by Industry Task Force II on 2,4-D Research Data, Indianapolis, IN, USA (as cited in: JMPR, 1996).
Schultze, G.E. (1991b). Subchronic toxicity study in rats with 2,4-D acid. Unpublished report No. 2184-116 from Hazleton Laboratories America, Inc., Vienna, VA, USA. Submitted to WHO by Industry Task Force II on 2,4-D Research Data, Indianapolis, IN, USA (as cited in: JMPR, 1996).
Semchuk, K.M., Love, E.J. and Lee, R.G. (1992). Parkinson's disease and exposure to agriculture work and pesticide chemicals. Neurology, 42(7): 1328-1335.
Serota, D.G. (1986). Combined chronic toxicity and oncogenicity study in rats with 2,4-D acid. Unpublished report. 2049. Hazleton Laboratories America, Vienna, VA, USA (as cited in Munro et al., 1992).
Serota, D., Burns, C., Burdock, G. et al. (1983a). Subchronic toxicity study in rats-2,4-dichlorophenoxyacetic acid (2,4-D). Unpublished study. Prepared by Hazleton Laboratories America, Inc., Project No. 2184-102 (as cited in JMPR, 1996).
Serota, D., Colpean, B. and Burdock, G.E.A. (1983b). Subchronic toxicity study in mice: 2,4-dichlorophenoxyacetic acid (2,4-D). Unpublished study. Prepared by Hazleton Laboratories America, Inc., Project No. 2184-100, as cited in JMPR, 1996.
Smeets, P.W.M.H., Medema, G.J., and van Dijk, J.C. (2009). The Dutch secret: how to provide safe drinking water without chlorine in the Netherlands. Drinking Water Engineering and Science, 2: 1-14.
Smith, F.A., Nolan, R.J., Hermann, E.A. and Ramsey, J.C. (1990). Pharmacokinetics of 2,4-dichlorophenoxyacetic acid (2,4-D) in Fischer 344 rats. Unpublished report No. 0697 from The Dow Chemical Company, submitted to WHO by Industry Task Force II on 2,4-D. Research Data, Indiana, MI, USA (as cited in JMPR, 1996).
Sørensen, S.R., Schultz, A., Jacobsen, O.S. and Aamand, J. (2006). Sorption, desorption and mineralization of the herbicides glyphosate and MCPA in samples from two Danish soil and subsurface profiles. Environ. Pollut., 141(1): 184-194.
Storck, F.R., Schmidt, C.K., Lange, F.T., Henson, J.W. and Hahn, K. (2010). Removal and fate of ECDs and PPCPs in bank filtration systems. ISBN - 978-1-60573-081-3. Water Research Foundation, Denver, Colorado and DVGW- Technologiezentrum Wasser, Karlsruhe, Germany.
Storck, F.R., Schmidt, C.K., Lange, F.T., Henson, J.W. and Hahn, K. (2012). Factors controlling micropollutant removal during riverbank filtration. J. Am. Water Works Assoc., 104(12): E643-E652.
Struger, J. and Fletcher, T. (2007). Occurrence of lawn care and agricultural pesticides in the Don River and Humber River watersheds (1998-2002). J. Great Lakes Res., 33(4): 887-905.
Struger, J., L'Italien, S. and Sverko, E. (2004). In-use pesticide concentrations in surface waters of the Laurentian Great Lakes, 1994-2000. J. Great Lakes Res., 30(3): 435-450.
Sturtz, Nelson, De Duffard, A.M.E., and Duffard, R. (2000). Detection of 2,4-dichlorophenoxy acetic acid (2,4-D) residues in neonates breast-fed by 2,4-D exposed dams. Neurotoxicology, 21:147-154.
Summers, R.S., Knappe, D.R.U. and Snoeyink, V.L. (2010). Adsorption of organic compounds by activated carbon. Chapter 14 in: Water quality and treatment: A handbook on drinking water. 6th edition. Edzwald, J.K. (ed.). McGraw-Hill, New York, New York.
Symons, J.M., Bradley Jr., L.C. and Cleveland, T.C. (2000). The drinking water dictionary. American Water Works Association, Denver, Colorado.
Szabo, J.C. and Rachunek, B.L. (1991). 2,4-D butoxyethyl ester: A 13-week dietary toxicity study in Fischer 344 rats. Unpublished report No. K-007722-015. Dow Chemical Company, Midland, MI, USA (as cited in JMPR, 1996).
Tan, Z., Zhou, J., Chen, H., Zou, Q., Weng, S., Luo, T. and Tang, Y. (2016). Toxic effects of 2,4-dichlorophenoxyacetic acid on human sperm function in vitro. J. Toxicol. Sci., 41(4): 543-549.
Tanner, C.M., Webster Ross, G., Jewell, S.A., Hauser, R.A., Jankovic, J., Factor, S.A., Bressman, S., Deligtisch, A., Marras, C., Lyons, K.E., Bhudhikanok, G.S., Roucoux, D.F., Meng, C., Abbott, R.D. and Langston, J.W. (2009). Occupation and risk of parkinsonism: A multicenter case-control study. Arch. Neurol., 66(9): 1106-1113.
Tayeb, W., Nakbi, A., Trabelsi, M., Miled, A. and Hammami, M. (2012). Biochemical and histological evaluation of kidney damage after sub-acute exposure to 2,4-dichlorophenoxyacetic herbicide in rats: involvement of oxidative stress. Toxicol. Mech. Methods, 22(9): 696-704.
Tayeb, W., Nakbi, A., Cheraief, I., Miled, A. and Hammami, M. (2013). Alteration of lipid status and lipid metabolism, induction of oxidative stress and lipid peroxidation by 2,4-dichlorophenoxyacetic herbicide in rat liver. Toxicol. Mechan. Methods, 23(6): 449-458.
Timchalk, C. (2004). Comparative inter-species pharmacokinetics of phenoxyacetic acid herbicides and related organic acids: Evidence that the dog is not a relevant species for evaluation of human health risk. Toxicology, 200(1): 1-19.
Timchalk, C., Dryzga, M. and Brzak, K.A. (1990). 2,4-Dichlorophenoxy-acetic, tissue distribution and metabolism of (carbon 14)-labeled 2,4-dichlorophenoxyacetic acid in Fischer 344 rats: Final report: Lab project number: K-2372-47. Unpublished study prepared by the Dow Chemical Company, MI, USA (as cited in US EPA, 2005).
Troudi, A., Ben Amara, I., Samet, A.M. and Zeghal, N. (2012). Oxidative stress induced by 2,4-phenoxyacetic acid in liver of female rats and their progeny: Biochemical and histopathological studies. Environ. Toxicol., 27(3): 137-145.
Tuduri, L., Harner, T., Blanchard, P., Li, Y., Poissant, L., Waite, D.T., Murphy, C. and Belzer, W. (2006). A review of currently used pesticides (CUPs) in Canadian air and precipitation. Part 2: Regional information and perspectives. Atmos. Environ., 40(9): 1579-1589.
US EPA (1991). National primary drinking water regulations-Synthetic organic chemicals and inorganic chemicals; monitoring for unregulated contaminants; national primary drinking water regulations implementations; national secondary drinking water regulations; Final rule. Fed. Reg., 56(20): 3526-3559.
US EPA (1992). Determination of chlorinated acids in water by high performance liquid chromatography with a photodiode array ultraviolet detector revision 1.0. National Exposure Research Laboratory, Office of Research and Development, US Environmental Protection Agency, Cincinnati, Ohio (EPA/600/R-92-129).
US EPA (1995a). Determination of chlorinated acids in water by gas chromatography with an electron capture detector revision 4.1. National Exposure Research Laboratory, Office of Research and Development, US Environmental Protection Agency, Cincinnati, Ohio (EPA/600/4-88-039).
US EPA (1995b). Determination of chlorinated acids in water using liquid-solid extraction and gas chromatography with an electron capture detector revision 1.1. National Exposure Research Laboratory, Office of Research and Development, US Environmental Protection Agency, Cincinnati, Ohio (EPA/600/R-95-131).
US EPA (1996). Determination of chlorinated acids in water by liquid-liquid extraction, derivation and gas chromatography with electron capture detector revision 1.0. National Exposure Research Laboratory, Office of Research and Development, US Environmental Protection Agency, Cincinnati, Ohio (EPA 815-R-00-014).
US EPA (2000). Determination of chlorinated acids in drinking water by liquid-liquid microextraction, derivation and fast gas chromatography with electron capture detector revision 1.0. National Exposure Research Laboratory, Office of Research and Development, US Environmental Protection Agency, Cincinnati, Ohio (EPA/815/B-00-001).
US EPA (2005). Registration eligibility decision for 2,4-D. List A. Case 0073. EPA 738-R-05-002. Available at: https://www3.epa.gov/pesticides/chem_search/reg_actions/reregistration/red_PC-030001_1-Jun-05.pdf.
US EPA (2007). 2,4-D, 2,4-DP, and 2,4-DB; Decision not to initiate special review. Fed. Reg., 72(152): 44510-44511.
US EPA (2009a). Analytical feasibility support document for the second six-year review of existing National Primary Drinking Water Regulations. Office of Water, US Environmental Protection Agency, Cincinnati, Ohio (EPA 815-B-09-003).
US EPA (2009b). Water treatment technology feasibility support document for chemical contaminants for the second six-year review of National Primary Drinking Water Regulations. Office of Water, US Environmental Protection Agency, Cincinnati, Ohio (EPA 815-B-09-007).
US EPA (2010). National primary drinking water regulations; Announcement of the results of EPA's review of existing drinking water standards and request for public comments and/or information on related issues. Fed. Reg., 75(59):15500-15503.
US EPA (2016). Analytical methods approved for drinking water compliance monitoring of organic contaminants. Office of Water, US Environmental Protection Agency, Cincinnati, Ohio (EPA 815-B-14-003).
University of Hertfordshire (2018). PPDB: Pesticide Properties Database. 2,4-D (Ref: L 208). Hertfordshire, U.K.
van der Aa, L.T.J., Kolpa, R.J., Rietveld, L.C. and van Dijk, J.C. (2012). Improved removal of pesticides in biological granular activated carbon filters by pre-oxidation of natural organic matter. J. Water Supply Res. Technol. AQUA, 61(3): 153-163.
van Ravenzwaay, B., Hardwick, T.D., Needham, D., Pethen, S. and Lappin, G.J. (2003). Comparative metabolism of 2,4-dichlorophenoxyacetic acid (2,4-D) in rat and dog. Xenobiotica, 33(8): 805-821.
Verstraeten, I.M., and Heberer, T. (2002). Organic chemical removal issues. Chapter 17 in: Riverbank filtration: Improving source-water quality. Ray, C., Melin, G. and Linsky, R.B. (eds.). Springer, Kluwer Academic Publishers. Netherlands. pp. 321-330.
Wafa, T., Amel, N., Issam, C., Imed, C., Abdelhedi, M. and Mohamed, H. (2011). Subacute effects of 2,4-dichlorophenoxyacetic herbicide on antioxidant defense system and lipid peroxidation in rat erythrocytes. Pestic. Biochem. Physiol., 99(3): 256-64.
Wester, R.C., Melendres, J., Sedik, L., Maibach, H. and Riviere, J.E. (1998). Percutaneous absorption of salicylic acid, theophylline, 2, 4-dimethylamine, diethyl hexyl phthalic acid, and p-aminobenzoic acid in the isolated perfused porcine skin flap compared to man in vivo. Toxicol. Appl. Pharmacol., 151: 159-165.
Westerhoff, P., Yoon, Y., Snyder, S. and Wert, E. (2005). Fate of endocrine-disruptor, pharmaceutical, and personal care product chemicals during simulated drinking water treatment processes. Environ. Sci. Technol., 39(17): 6649-6663.
WHO (2003). 2,4-D in Drinking-water: Background document for development of WHO Guidelines for Drinking-water Quality. World Health Organization, Geneva, Switzerland. Available at: www.who.int/water_sanitation_health/ dwq/chemicals/24D.pdf
WHO (2011). Guidelines for drinking-water quality. 4th ed. World Health Organization, Geneva, Switzerland. ISBN 978 92 4 154815 1.
WHO (2012). Water safety planning for small community water supplies. World Health Organization, Geneva, Switzerland. Available at: http://www.who.int/water_sanitation_health/publications/small-commwater_supplies/en/
WHO (2017). Guidelines for drinking-water quality. 4th ed. Incorporating the first addendum. World Health Organization, CC BY-NC-SA 3.0 IGO, Geneva, Switzerland. Available at: www.who.int/water_sanitation_health/ publications/drinking-water-quality-guidelines-4-including-1st-addendum/en/
Wiklund, K., Holm, L.E. and Dich, J. (1987). Soft tissue sarcoma risk among agricultural and forestry workers in Sweden. Chemosphere, 16(8-9): 2107-2110.
Wols, B.A. and Hofman-Caris, C.H.M. (2012). Review of photochemical reaction constants of organic micropollutants required for UV advanced oxidation processes in water. Water Res., 46: 2815-2827.
Woodruff, R.C., Phillips, J.P. and Irwin, D. (1983). Pesticide-induced complete and partial chromosome loss in screens with repair-defective females of Drosophila melanogaster. Environ. Mutagen., 5(6): 835-846.
Woudneh, M.B., Lloyd, B.J. and Stevenson, D. (1996). Removal of herbicides by biological filters. In: Advances in slow sand/alternative biofiltration. Graham, N. and Collins, M.R. (eds.), John Wiley & Sons. Chichester, United Kingdom. pp. 211-221.
Woudneh, M.B., Lloyd, B.J. and Stevenson, D. (1997). The behaviour of 2,4-D as it filters through slow sand filters. J. Water Supply Res. Technol. AQUA, 46(3): 144-149.
Xiong, F. and Graham, N.J.D. (1992). Rate constants for herbicide degradation by ozone. Ozone Sci. Eng., 14: 283-301.
Yao, C.C.D. and Haag, W.R. (1991). Rate constants for direct reactions of ozone with several drinking water contaminants. Wat. Res. 25(7): 761-773.
Yao, Y., Harner, T., Blanchard, P., Tuduri, L., Waite, D., Poissant, L., Murphy, C., Belzer, W., Aulagnier, F. and Sverko, E. (2008). Pesticides in the atmosphere across Canadian agricultural regions. Environ. Sci. Technol., 42: 5931-5937.
Zahm, S.H., Weisenburger, D.D., Babbitt, P.A., Saal, R.C., Vaught, J.B., Cantor, K.P. and Blair, A. (1990). A case-control study of non-Hodgkin's lymphoma and the herbicide 2,4-dichlorophenoxyacetic acid (2,4-D) in eastern Nebraska. Epidemiology, 1(5): 349-356.
Zearley, T.L. and Summers, R.S. (2012). Removal of trace organic micropollutants by drinking water biological filters. Environ. Sci. Technol., 46: 9412-9419.
Zearley, T.L. and Summers, R.S. (2015). MIB and 2,4-D removal by biofilters during episodic loading. J. Am. Water Works Assoc., 107(12): E666-E673.
Zimmering, S., Mason, J.M., Valencia, R. and Woodruff, R.C. (1985). Chemical mutagenesis testing in Drosophila. II. Results of 20 coded compounds tested for the National Toxicology Program. Environ. Mutagen., 7(1): 87-100.
Appendix A: List of abbreviations
- 2,4-D
- 2,4-dichlorophenoxyacetic acid
- ADI
- acceptable daily intake
- a.i.
- active ingredient
- ALP
- alkaline phosphatase
- ALT
- alanine transaminase
- ANSI
- American National Standards Institute
- AOP
- advanced oxidation process
- APHA
- American Public Health Association
- AST
- aspartate transaminase
- AWWA
- American Water Works Association
- BUN
- blood urea nitrogen
- BVs
- bed volumes
- bw
- body weight
- CAF
- composite assessment factor
- CAS
- chemical abstracts service
- CI
- confidence interval
- DL
- detection limit
- DMA
- dimethylamine
- DNA
- deoxyribonucleic acid
- DOC
- dissolved organic carbon
- EBCT
- empty bed contact time
- ECD
- electron capture detector
- EPA
- Environmental Protection Agency (US)
- EU
- European Union
- GAC
- granular activated carbon
- GD
- gestation day
- GC
- gas chromatography
- H2O2
- hydrogen peroxide
- HBV
- health-based value
- IARC
- International Agency for Research on Cancer
- IPA
- isopropylamine
- LD50
- median lethal dose
- LLMED
- liquid-liquid microextraction, derivatization
- LOD
- limit of detection
- MAC
- maximum acceptable concentration
- MCL
- maximum contaminant level
- MCLG
- maximum contaminant level goal
- MDL
- method detection limit
- NOAEL
- no-observed-adverse-effect level
- NOEL
- no-observed-effect level
- NOM
- natural organic matter
- NSF
- NSF International
- O3
- ozone
- OR
- odds ratio
- PAC
- powdered activated carbon
- PMRA
- Pest Management Regulatory Agency
- PND
- postnatal day
- SCC
- Standards Council of Canada
- SCE
- sister chromatid exchange
- T4
- thyroxin
- TIPA
- triisopropanolamine
- UV
- ultraviolet
- VOCs
- volatile organic chemicals
- WHO
- World Health Organization
Appendix B: Canadian water quality data
Jurisdiction (year sampled) |
No. detects/ samples |
MDL (ng/L) |
Range (ng/L) | 25th percentile (ng/L) | Mean (ng/L) | Median (ng/L) | 75th percentile (ng/L) | |
---|---|---|---|---|---|---|---|---|
Min | Max | |||||||
Tap water | ||||||||
AB, SK, MB – rural communities (2004–2005) |
?/28 | N/A | 10.50 | 589.00 | N/A | 81.40 | N/A | N/A |
Surface Water | ||||||||
BC – Lower Fraser Valley and Okanagan Basin (2003–2005) |
59/92 | 0.5 | < 0.5 | 1230 | 0.680 | N/A | 2.720 | 22.95 |
BC – Lower Fraser Valley (2003–2005) |
N/A | N/A | 0.62 | 1230 | N/A | N/A | N/A | N/A |
BC – Okanagan Basin (2003–2005) |
N/A | N/A | 0.59 | 41.8 | N/A | N/A | N/A | N/A |
ON (2003) | 156/160 | 0.47 | 1.3 | 2850 | 7.06 | N/A | 46.50 | 114.50 |
ON (2004) | 184/228 | 0.47 | 0.71 | 8240 | 1.84 | N/A | 12.75 | 61.95 |
ON (2005) | 148/183 | 0.47 | 0.92 | 4220 | 1.59 | N/A | 10.8 | 76.5 |
QC (2003) | 5/51 | 20 | < 20 | 120 | N/A | N/A | N/A | N/A |
QC (2004) | 25/70 | 10–20 | < 10 | 190 | N/A | N/A | N/A | N/A |
QC (2004)Footnote b | 23/69 | 3–50 | < 3 | 130 | N/A | N/A | N/A | N/A |
QC (2005) | 13/59 | 20 | < 20 | 340 | N/A | N/A | N/A | N/A |
NB (2003–2005) | 0/57 | 100 | N/A | N/A | N/A | N/A | N/A | N/A |
PEI (2003–2005) | 0/82 | 100 | N/A | N/A | N/A | N/A | N/A | N/A |
NS (2003–2005) | 0/48 | 100 | N/A | N/A | N/A | N/A | N/A | N/A |
Rivers | ||||||||
AB, SK, MB – 8 sites (2003) |
59/64 | 0.47 | < 0.47 | 457 | 18.50 | N/A | 46.00 | 65.00 |
Reservoir Water | ||||||||
AB, SK, MB – 15 sites (2003–2004) |
205/206 | 0.47 | < 0.47 | 1850 | 23.85 | N/A | 66.30 | 129.00 |
Runoff | ||||||||
BC – Okanagan Basin (2003–2005) |
N/A | N/A | 0.61 | 445 | N/A | N/A | N/A | N/A |
Groundwater | ||||||||
BC – Lower Fraser Valley (2003–2005) |
N/A | N/A | N/A | 5.01 | N/A | N/A | N/A | N/A |
BC – Lower Fraser Valley (2003–2005)Footnote a |
N/A | N/A | 0.001 | 4.93 | N/A | N/A | N/A | N/A |
MDL = method detection limit; NA = not available
Note: Adapted from Environment Canada, 2011 |
Footnotes
- Footnote 1
-
The terms ALT and SGPT (serum glutamic pyruvic transaminase) refer to the same parameter and are interchangeable.
- Footnote 2
-
The terms AST and SGOT (serum glutamic oxaloacetic transaminase) refer to the same parameter and are interchangeable.
Page details
- Date modified: