Manganese in Drinking Water
Document for Public Consultation
Prepared by the Federal-Provincial-Territorial Committee on Drinking Water
Consultation period ends August 5, 2016
Table of contents
- Purpose of consultation
- Part I. Overview and Application
- Part II. Science and Technical Considerations
- 4.0 Identity, use and sources in the environment
- 5.0 Exposure
- 6.0 Analytical methods
- 7.0 Treatment technology and distribution system considerations
- 7.1 Manganese chemistry and treatment
- 7.2 Municipal scale treatment
- 7.3 Distribution system
- 7.4 Residential scale treatment
- 8.0 Kinetics and metabolism
- 9.0 Health effects in humans
- 10.0 Health effects on experimental animals
- 11.0 Mode of action
- 12.0 Classification and assessment
- 13.0 Rationale
- 14.0 References
- Appendix A: List of acronyms
- Appendix B: Provincial/territorial cost estimates

Download the entire report
(PDF format, 882 KB, 116 pages)
Related Topics
Purpose of consultation
The Federal-Provincial-Territorial Committee on Drinking Water (CDW) has assessed the available information on manganese with the intent of updating the current drinking water guideline and guideline technical document on manganese in drinking water. The purpose of this consultation is to solicit comments on the proposed guideline, on the approach used for its development and on the potential economic costs of implementing it, as well as to determine the availability of additional exposure data.
The existing guideline on manganese, last updated in 1987, established an aesthetic objective (AO) of 0.05 mg/L (50 µg/L), based on treatment limitations as well as taste and staining of laundry and plumbing fixtures. This expanded and updated document proposes both a maximum acceptable concentration (MAC) and an aesthetic objective for total manganese in drinking water. The proposed MAC of 0.1 mg/L (100 µg/L) is based on neurological effects observed in rodents. Similar effects have been observed in epidemiological studies conducted in children. The proposed AO of 0.02 mg/L (20 µg/L) would minimize the occurrence of discoloured water complaints and improve consumer confidence in drinking water quality. The document provides updated data and information related to exposure to manganese in Canada and to analytical methods and treatment technologies available at the municipal and residential scales.
The CDW has requested that this document be made available to the public and open for comment. Comments are appreciated, with accompanying rationale, where required. Comments can be sent to the CDW Secretariat via email at water_eau@hc-sc.gc.ca. If this is not feasible, comments may be sent by mail to the CDW Secretariat, Water and Air Quality Bureau, Health Canada, 3rd Floor, 269 Laurier Avenue West, A.L. 4903D, Ottawa, Ontario K1A 0K9. All comments must be received before August 5, 2016.
Comments received as part of this consultation will be shared with the appropriate CDW member, along with the name and affiliation of their author. Authors who do not want their name and affiliation shared with their CDW member should provide a statement to this effect along with their comments.
It should be noted that this guideline technical document on manganese in drinking water will be revised following evaluation of comments received, and a drinking water guideline will be established, if required. This document should be considered as a draft for comment only.
Part I. Overview and Application
1.0 Proposed guideline
A maximum acceptable concentration (MAC) of 0.1 mg/L (100 µg/L) is proposed for total manganese in drinking water. An aesthetic objective (AO) of 0.02 mg/L (20 µg/L) is also proposed for total manganese in drinking water.
2.0 Executive summary
Manganese occurs naturally in the environment and is widely distributed in air, water and soil. It is not found in the elemental form in the environment, but can exist in several oxidation states. Manganese may be present in water in the environment from natural sources (rock and soil weathering) or as a result of human activities (such as mining, industrial discharges and landfill leaching). Manganese is used in various industries, including in the steel industry, in the manufacture of various products (e.g., fireworks, dry-cell batteries, fertilizers, fungicides and cosmetics and paints). In its permanganate form, it is also used as an oxidizing agent in the treatment of drinking water.
This guideline technical document reviews and assesses all identified health risks associated with manganese in drinking water. It incorporates new studies and approaches and takes into consideration the availability of appropriate treatment technology. Based on this review, the proposed drinking water guideline for manganese is a maximum acceptable concentration (MAC) of 0.1 mg/L (100 µg/L), based on infants, the most sensitive population.
During its fall 2015 meeting, the Federal-Provincial-Territorial Committee on Drinking Water reviewed the guideline technical document on manganese and gave approval for this document to undergo public consultation.
2.1 Health effects
Manganese is an essential element for humans. Deficiency is considered unlikely in Canada, as adequate amounts are obtained from food. A non-cancer endpoint was chosen for this assessment as available studies are not adequate to support a link between manganese and cancer. Some studies in humans suggest an association between manganese in drinking water and neurological effects in children; however, they can only be used to support the choice of the key health effect. The effects observed in children are consistent with the neurological effects reported in the key animal studies used to establish the proposed MAC.
2.2 Aesthetic considerations
Concerns regarding the presence of manganese in drinking water are often related to consumer complaints regarding discoloured water. The proposed AO of 0.02 mg/L (20 µg/L) would minimize the occurrence of discoloured water complaints and improve consumer confidence in drinking water quality.
2.3 Exposure
Manganese occurs naturally and is widely distributed in the environment. Canadians can be exposed to manganese through its presence in air, food, consumer products, soil and drinking water, with food being the main source of exposure. However, manganese is more readily absorbed from drinking water than when it is ingested with food. Levels of manganese in fresh water in Canada are usually below 0.1 mg/L, with some spikes reaching into the milligrams per litre range. Higher levels can occur in acidic groundwater and with industrial discharges. Manganese is generally more prevalent in ground waters than in surface waters. Intake of manganese from drinking water is not expected through either skin contact or inhalation.
2.4 Analysis and treatment
There are several analytical methods available for the analysis of total manganese in drinking water at levels well below the proposed MAC and AO. Total manganese includes both the dissolved and particulate forms of manganese in a water sample. Therefore, if the two forms are measured separately, the two concentrations must be added before comparison with the MAC and AO.
There are various methods available to decrease levels of manganese in drinking water to below the proposed MAC. The choice of an appropriate method will depend on the form of manganese present in the source water. Low levels of manganese in source or treated water may accumulate in the distribution system and periodically lead to high levels of manganese at the tap. The accumulation of manganese oxides in the distribution system may also cause the release of other contaminants into the distributed water. Most well operated and optimized treatment plants can achieve manganese concentrations of 0.015 mg/L or less in the treated water, which would minimize the accumulation of manganese and ithe associated potential release of manganese or other contaminants in the distributed water. This would help prevent the presence of manganese at consumers’ taps above the AO and reduce both consumer complaints related to discoloured water and the potential for higher manganese concentrations at consumers’ taps (which could be above the proposed MAC).
There are several treatment technologies that can be effective for manganese removal at the residential scale; however there are currently no treatment units certified specifically for that purpose.
3.0 Application of the guideline
Note: Specific guidance related to the implementation of drinking water guidelines should be obtained from the appropriate drinking water authority in the affected jurisdiction.
3.1 Monitoring
3.1.1 Source water characterization
Water sources should be characterized to determine if manganese is present. This should include sampling during periods when manganese is mostly likely to be elevated in surface waters such as during thermal stratification in the summer and lake turnover in the fall. While manganese concentrations in groundwater are less likely to fluctuate between seasons, large variations have been observed between wells located in close proximity to each other. Therefore, all wells in a well field should be characterized. Monitoring of surface water should be conducted quarterly with weekly monitoring during summer/fall in lakes and reservoirs subject to stratification and/or large fluctuations in manganese concentrations. Groundwater sources should be monitored semi-annually. Authorities may consider reduced monitoring when it has been demonstrated that manganese is present at concentrations equal to or below 0.02 mg/L in the source water and/or appropriate treatment is in place.
3.1.2 Treatment plant
Utilities that treat their water to remove manganese need to conduct frequent monitoring of raw and treated water, in order to make necessary process adjustments and to ensure that treatment processes are effectively removing manganese concentrations below the MAC and the AO. The frequency of monitoring of finished water will depend on historical knowledge of manganese fluctuations in the raw water and the type of treatment processes in place. For example, surface water treatment plants where manganese concentrations fluctuate and oxidation and filtration are used for treatment may need to monitor daily. Monitoring within the treatment plant, at key treatment steps, may be needed if a utility is having difficulty controlling manganese concentrations in the treated water. Appropriate filtration should be conducted on a portion of samples collected to determine the particulate and dissolved manganese components. This is important to determine the type of treatment needed and to assess treatment plant performance. Utilities that are experiencing difficulties controlling manganese in treated water, and that are directly oxidizing manganese using potassium permanganate, chlorine dioxide or ozone, may also consider quantifying the colloidal manganese fraction of selected samples within the treatment train. In many cases, process monitoring within a treatment plant can be conducted using colorimetric methods to reduce analytical costs.
3.1.3 Distribution system
Since manganese can accumulate and release in distributions systems, monitoring within the distribution system and at consumers’ taps should also be conducted for systems where manganese is or was historically present in the source water. This will help ensure that operations and maintenance are adequate to maintain manganese concentrations in the distribution system below the AO. Since manganese releases tend to be sporadic events, it is difficult to establish a practical routine monitoring programme that could effectively detect manganese in tap water due to manganese release within the distribution system. However the risk factors associated with both distribution system manganese accumulation and release could be used as indicators of when (event-based) and where to monitor for manganese releases. Event-based monitoring may be needed during conditions where risk of release is increased, such as following any hydraulic disturbances (e.g., main breaks or hydrant flushing) to the system or changes in water chemistry (e.g., changes to pH, temperature, source water type or uncontrolled source water blending, chlorine residual, or uncontrolled disinfectant blending). Distribution system sampling locations would ideally be located where there are both increased risk factors for manganese accumulation (e.g., proximity to water treatment plant, pipe materials, biofilm) and event-based release risk factors. Monitoring should also be conducted during any discoloured water event, although the absence of discoloured water should not be interpreted as the absence of a manganese release. Monitoring for manganese should be done in conjunction with other metals that can co-occur in the distribution system and have been shown to release with manganese (e.g., iron, arsenic, lead). Utilities that undertake preventive measures with stable hydraulic, physical and water quality conditions and have baseline data indicating that manganese does not occur in the system may conduct less frequent monitoring.
3.1.4 Compliance
Monitoring for manganese should be conducted primarily at consumers’ taps, with a portion of samples collected from sites that are located in close proximity to the treatment plant. At a minimum, routine monitoring is recommended to be conducted quarterly. In addition, event based monitoring should be conducted during conditions where the risk of release is increased or when discolouration of water has been reported.
3.1.5 Residential
Homeowners with private wells are also encouraged to have their water tested for manganese once a year to ensure that the concentration in their water supply is below the MAC. In addition, homeowners with private wells using point-of-entry or point-of-use treatment devices should conduct routine testing on both the water entering the treatment device and the treated water to verify that the treatment device is effective.
Part II. Science and Technical Considerations
4.0 Identity, use and sources in the environment
Manganese (CAS Registry No. 7439-96-5) is a group VIIB transition metal that often naturally co-exists with iron in geologic deposits and soils, and corrodes under atmospheric conditions. Manganese has a boiling point of 1,962°C and a melting point of 1,244°C. It has a vapor pressure of 1 mm Hg at 1292°C (summarized in U.S. EPA, 2004; ATSDR, 2012). Manganese exists in both organic and inorganic forms, which have different physico-chemical characteristics. Manganese is an essential element for many organisms including humans, and is present in many foods (IOM, 2001).
Manganese occurs naturally in the environment (widely distributed in air, water, soil) and constitutes 0.1% of the Earth’s crust; however, it is not found in the elemental form (Barceloux, 1999; Kohl and Medlar, 2006; WHO, 2011). The concentration in various media varies widely depending on the environmental conditions and proximity to anthropogenic sources such as ferroalloy industry facilities (U.S. EPA, 2004). Manganese can have various oxidation states, (the most common being +2, +4, and +7), and can form a large variety of complexes by combining with other elements such as oxygen, sulphur and chlorine, as well as carbonates and silicates (Stokes et al., 1988; ATSDR, 2012). The most abundant minerals found in soil are pyrolusite (manganese dioxide), rhodochrosite (manganese carbonate [MnCO3]), and rhodonite (manganese silicate) (Stokes et al., 1988; IPCS, 1999; Kohl and Medlar, 2006). Mn(IV) oxide is the most abundant form found in manganese ores (Kohl and Medlar, 2006). Only a dozen of some of the 300 minerals containing manganese are considered significant geologic ore deposits to support manganese mining (International Manganese Institute, 2014).
Studies have been conducted examining the visual and taste thresholds of manganese in drinking water (Cohen et al., 1960; Sain et al., 2014). Sain et al. (2014) tested the visual and taste thresholds for dissolved Mn(II) and particulate Mn(IV) using one-in-five, one-in-three, and triangle tests for concentrations between 0.005 and 506 mg/L. Results indicated that the population best estimate taste threshold of Mn(II) from manganese sulfate was 101 mg/L although this was likely influenced by both the sulfate and Mn(II) taste contributions. Logistic regression of the data indicated that the 50% population taste threshold for Mn(II) was 75.4 mg/L. Taste threshold testing for Mn(IV) was limited due to discolouration of the water; however, it was reported that 0.05 mg/L of Mn(IV) could not be distinguished from distilled water. The visual threshold testing found that dissolved Mn(II) is colourless and remains visually undetectable at concentrations as high as 506 mg/L. In contrast, particulate Mn(IV) was visually detectable by 100% of participants at a concentration of 0.005 mg/L. The authors concluded that a manganese concentration of 0.01–0.02 mg/L is an appropriate limit to protect against the discolouration of water. This is supported by numerous studies of drinking water systems that have reported that consumer complaints about discoloured water and/or staining of laundry occur at manganese concentrations above 0.02 mg/L (Sly et al., 1990; Casale et al., 2002; Kohl and Medlar, 2006). Based on this information it has been suggested that drinking water guidelines for manganese established at 0.05 mg/L should be lowered to 0.02 mg/L to minimize the occurrence of discoloured water complaints and improve consumer confidence in drinking water quality (Dietrich and Burlingame, 2015).
Surface and ground water sources of manganese can be natural (from rock and soil weathering) and anthropogenic (from industrial discharges, mining activities and landfill leaching) (Stokes et al., 1988; Kohl and Medlar, 2006; Ljung and Vahter, 2007). Physico-chemical properties of the local environment (e.g., organic carbon content, cation exchange capacity, pH, Eh, mineral and particulate content) influence the manganese speciation and aqueous solubility, which in turn influences the concentration in ground and surface waters (Stokes et al., 1988; Kohl and Medlar, 2006). In surface water, manganese occurs in particulate, colloidal, organic and dissolved forms. Most inorganic manganese salts are soluble to some extent in water. Manganese carbonate has lower solubility (0.065 g/L) than the halide salt, but is the major contributor to the dissolved manganese found in water (IPCS, 1999; Luo and Millero, 2003). More soluble forms include manganese chloride (MnCl2), potassium permanganate (KMnO4) and manganese sulphate (MnSO4), which have solubilities of 72.3, 6.38 and 52 g/L, respectively (as summarized in CRC, 1983). In water, the most common oxidation states are Mn(II), Mn(IV) and Mn(VII) (Stokes et al., 1988; ATSDR, 2012; Rumsby et al., 2014). Mn(III), Mn(V) and Mn(VI) are not stable in neutral solutions. Acidic media, lower reducing environments and the presence of nitrates, sulphates and chlorides favour the reduction of Mn(III) and Mn(IV) mineral compounds to the more stable and soluble Mn(II) form and the formation of manganese sulfate and manganese chloride (Stokes et al., 1988; WHO, 2004; Kohl and Medlar, 2006; ATSDR, 2012). In alkaline (pH> 8–9) and oxidizing conditions (in the presence of chlorine, ozone, etc.), conversion of Mn(II) to Mn(IV) will occur, with manganese precipitating and forming insoluble minerals of Mn(IV) compounds (WHO, 2004). Hence, Mn(IV) is insoluble, but can be found in the particulate state of water (Kohl and Medlar, 2006). The oxidation-reduction cycle can be perpetrated abiotically or biotically (IPCS, 1999; WHO, 2004).
The concentration of manganese in soil varies, and is more prevalent in metamorphic and sedimentary rock (Stokes et al., 1988). The form of manganese compounds in the soil depends mainly on the soil and water pH and reduction potential; however, soil mineralogy, oxidative microbial activity and organic matter content are also important related factors (WHO, 2004, 2011). For example, the formation of soluble manganese is favoured by an acidic pH and reducing conditions, such as caused by acid rain and during decomposition of organic matter (Kohl and Medlar, 2006). The soil retention of manganese results from the cation exchange capacity of the soil, forming insoluble manganese oxides and hydroxides, and from the adsorption to oxides and hydroxides (WHO, 2004).
The main sources of particulate manganese in the atmosphere stem from industrial activities including iron and steel-production plants, power plants, coke ovens, and dust from mining operations. Natural sources of manganese can also increase atmospheric levels through volcanic eruptions, forest fires, ocean spray and soil erosion (Stokes et al., 1988; IPCS, 1999; U.S. EPA, 2004). Manganese particulates released into the atmosphere, such as manganese dioxide (MnO2) and manganese tetroxide (Mn3O4), are small and carried over long distances before deposition onto soil or surface waters.
The reduction-oxidation properties of manganese allow its usage in various industries (Kohl and Medlar, 2006). The steel industry represents the principal use of manganese, mainly to improve mechanical properties of stainless steel and ferromanganese and aluminum alloys (Barceloux, 1999; U.S. EPA, 2004; ATSDR, 2012). Manganese dioxide and chloride are used by industries in dry-cell batteries, fireworks, gasoline, agriculture, leathers and textiles, and as a contrasting agent in magnetic resonance imaging (IPCS, 1999; International Manganese Institute, 2014). Manganese sulphate is used in fertilizers and some fungicides. Manganese is also used in cosmetics and paints for its coloring properties. The permanganate ion (MnO4–) is used by drinking water treatment plants as an oxidizing agent to remove iron and other contaminants. Use of the gasoline additive methylcyclopentadienyl manganese tricarbonyl declined dramatically in 2004 in Canada due to voluntary measures taken by national petroleum refiners (Walsh, 2007).
5.0 Exposure
Canadians can be exposed to manganese through its presence in air, food, consumer products, soil and drinking water; however, the main source of exposure is through diet (Barceloux, 1999). Manganese is an essential element, being a cofactor for enzymes involved in antioxidant defences, growth, and metabolism, and, as such, has daily recommended intakes set by international organizations (IPCS, 1999; IOM, 2001). The bioavailability of Mn from drinking water (in a fasted state) has been acknowledged to be greater than from food in both published literature and in other risk assessments (Ruoff et al., 1995; IRIS, 2002; U.S. EPA, 2004; Bouchard et al., 2011).
5.1 Water
Typical levels of manganese in fresh water are in the range of 1 to 200 μg/L, as either dissolved Mn(II) or as particulate manganese oxides, hyroxides and carbonates. Higher levels can occur in groundwater or surface water that are acidic, have a low Eh (oxidation/reduction potential) or are affected by industrial discharges. This is reflected by Canadian data, which indicates that most drinking water contains less than 100 μg/L of Mn, with a few cases where water manganese levels may reach thousands of micrograms per litre. Generally, manganese is more prevalent in groundwater supplies than in surface water supplies because of the increased reducing conditions of the former (WHO, 2011). The National Drinking Water Survey reported manganese concentrations in drinking water in various locations distributed across Canada (Health Canada, 2014a). Treated drinking water points in the distribution systems were sampled and analysed for dissolved manganese in all provinces and territories (n = 65). In 2009, 39% of the samples were below the limit of detection (2 μg/L) and 63.2% in 2010. The means were 16.1 μg/L in 2009 and 10.8 μg/L in 2010; and 75% of the measurements were under 11 μg/L in 2009 and under 8 μg/L in 2010. There were only 2 measurements over 100 μg/L in both years (maximum value was 440 μg/L in 2009 and 160.0 μg/L in 2010).
In British Columbia, the Ministry of Environment reported that manganese was monitored in various locations including both surface and groundwater between 1991 and 2014 (British Columbia Ministry of Health, 2014). Total manganese (chemical extraction) was equal or less than 5 μg/L in 288/3,593 samples (8%), the average manganese was 96.1 μg/L, the median was 8 μg/L and 417 samples were above 200 μg/L (12%). Dissolved manganese was equal or less than 5 μg/L in 4/155 samples (3%), the average manganese was 311 μg/L, the median was 8 μg/L, 20 samples were above 200 μg/L (13%) and 6 samples were above 10,000 μg/L (4%).
In Alberta, manganese levels were measured between 2003 and 2013 in two water treatment plants that received water from the Elbow and Bow rivers in Calgary, and in four of their distribution systems (North and South ends of Calgary) (Alberta Environment and Sustainable Resource Development, 2014). Dissolved and total manganese in untreated raw water (n = 2810) was not detected in 17 samples (less than 1%, detection limit 0.4–0.5 μg/L), the average was 8.7 μg/L, the median was 0.60 μg/L and 31 samples were above 50 μg/L (1%), with a maximum of 434.3 μg/L. In the treatment plant effluents (n = 2,175), dissolved and total manganese were not detected in 1,578 samples (72%, detection limit 0.4–0.5 μg/L), the average was 0.61 μg/L, the median was 0.5 μg/L and the maximum was 16.3 μg/L. In the distribution system (n = 1925), dissolved and total manganese was not detected in 1169 samples (59%), the average was 0.74 μg/L, the median was 0.5 μg/L and the maximum was 12.6 μg/L.
In Saskatchewan, treated drinking water samples were taken in the distribution system (95% of samples) and treatment plants between 2003 and 2014 (n = 2239 in 640 sites, detection limit 0.5–10 μg/L) (Saskatchewan Water Security Agency, 2014). In the distribution system, a mean of 143 μg/L, a median of 10 μg/L and a maximum of 8,440 μg/L were reported for total and dissolved Mn. Treated water had a mean of 229 μg/L, a median of 10 μg/L and a maximum of 3,510 μg/L.
In Manitoba, raw (n = 1,648) and treated (n = 1,586) water samples were taken between 2002 and 2012 (Manitoba Conservation and Water Stewardship, 2015). In raw water, a mean of 201 μg/L, a median of 25 μg/L, and a maximum of 9,870 μg/L were reported for total Mn, and 70% of the measurements were below 100 μg/L and 23% above 200 μg/L. Treated water had a mean of 71 μg/L, a median of 8 μg/L and a maximum of 5,150 μg/L, and 91% of the measurements were below 100 μg/L and 6% above 200 μg/L. The limits of detection were not provided.
In Ontario, levels of manganese in drinking water were reported for samples taken between 2009 and 2014 (Ontario Ministry of the Environment and Climate Change, 2014). In distribution systems (measured at treatment plants), manganese levels were at or below 0.1 μg/L in 153/1,330 samples (detection limit of 0.05–0.1), the mean was 4.4 μg/L, the median was 1.5 μg/L, 4 were above 100 μg/L, none above 200 μg/L and the maximum was 187 μg/L. In treated water (measured at the tap), manganese levels were at or below 0.1 μg/L in 222/1,700 samples, the mean was 5.8 μg/L, the median was 1.2 μg/L, 12 were above 100 μg/L, 2 were above 200 μg/L and the maximum was 442 μg/L. In raw water, manganese levels were at or below 0.1 μg/L in 98/2,105 samples, the mean was 23.5 μg/L, the median was 6.8 μg/L, 109 were above 100 μg/L, 51 were above 200 μg/L and the maximum was 838 μg/L.
In Quebec, levels of manganese in treatment plants and the distribution system with groundwater sources between 2010 and 2014 were reported (Ministère du Développement durable, de l’Environnement et de la Lutte contre les changements climatiques, 2014). The average manganese in raw water (n = 283) was 86.6 μg/L, the median was 4.3 μg/L, 36 samples (13%) were at or above 200 μg/L and the maximum was 4,100 μg/L (detection limit was 0.2 μg/L). The average manganese in treated water (n = 126) was 35.4 μg/L; the median was 2.9 μg/L; 22 samples (17%) were above 50 μg/L, and 7 samples were above 200 μg/L; and the maximum was 470.0 μg/L. The only manganese measurement in the distribution system was 68 μg/L. A study in Southwest Quebec reported levels between 0.5–71.1 μg/L (mean of 4.11 μg/L) for municipal sources (n = 262) (Baldwin et al., 1999). Wells had levels between 0.2–158.9 μg/L (n = 16; mean of 2.78 μg/L).
In New Brunswick, drinking water manganese was measured in Crown (all were from groundwater wells) and municipal water supply systems (ground and surface water) (New Brunswick Department of Health, 2014). Drinking water in Crown systems had levels of manganese at or below 5 μg/L in 957/1817 samples (53%), the mean was 88 μg/L, the median was 5 μg/L, 310 were above 200 μg/L (17%) and the maximum was 4,000 μg/L. In distribution system of municipal water, manganese levels were at or below 5 μg/L in 1,186/2,115 samples (56%), the mean was 75 μg/L, the median was 5 μg/L, 139 were above 200 μg/L (7%) and the maximum was 2,560 μg/L. In municipal groundwater sources 563/2,081 had levels of manganese at or below 5 μg/L (27%), the mean was 306 μg/L, the median was 53 μg/L, 694 were above 200 μg/L (33%) and the maximum was 4,400 μg/L. For municipal surface water sources, 154/490 had levels of manganese at or below 5 μg/L (31%), the mean was 172 μg/L, the median was 28 μg/L, 60 were above 200 μg/L (12%) and the maximum was 3,130 μg/L. Additionally, some information on the content of manganese in private wells (n = 10,571) was reported in geo-referenced samples in the New Brunswick Groundwater Chemistry Atlas 1994–2007 (New Brunswick Minister of Environment and Local Government, 2008). There were 7,811 samples above 5 μg/L (74%), 4,207 samples above 50 μg/L (40%) and the maximum concentration was 33,600 μg/L. The Sackville treatment plant reported a typical range of 1,500 to 2,500 μg of total Mn/L for raw water, and less than 50 μg/L for finished water concentration leaving the treatment plant.
In Newfoundland and Labrador, levels of manganese in drinking water were reported for samples taken between 2011 and 2014 (Newfoundland and Labrador Department of Environment and Conservation, 2014). All measurements were above the detection limit (10 μg/L). Tap water (n = 253) had a mean of 170.9 μg/L, a median of 120 μg/L and the maximum was 980 μg/L. Source water (n = 96) had a mean of 224.6 μg/L, median of 115 μg/L and the maximum was 2,400 μg/L.
In Yukon, levels of manganese in drinking water were reported for 21 groundwater sources taken in 2012, 2013 and 2014 (detection limit 1–50 μg/L) (Yukon Environmental Health Services, 2014). The mean manganese concentrations were 60.3 μg/L and 13.3 μg/L; and the medians were 20 μg/L and 6 μg/L in raw drinking water (n = 33) and in treated drinking water (n = 13), respectively. There were 11 samples above 100 μg/L (23.9%, all from raw water) and the maximum was 209 μg/L.
5.2 Food
Food is the main source of exposure to manganese, with grains, nuts and vegetables contributing to most of the intake. Average concentrations of manganese were estimated in various food items in the Canadian Total Diet Study (TDS) (Health Canada, 2009). High manganese concentrations (above 1.0 μg/g) were reported for grains (bread [3.5–19.1 μg/g], white flour [3.5–7.5 μg/g], rice [3.0–4.9 μg/g], cereals [1.0–25.9 μg/g] , popcorn [7.2–10.6 μg/g]), nuts (28.5 μg/g), peanut butter (13.1–16.8 μg/g), organ meats (3.1–4.0 μg/g), some fruits (blueberries [8.3–10.0 μg/g], canned pineapples [12.0–20.4 μg/g], bananas [1.7–4.6 μg/g]), vegetables (0.8–3.0 μg/g for beets, broccoli, cabbage, cauliflower, carrots, celery, lettuce, corn, cucumbers, onions, potatoes and 3.0–7.5 μg/g for spinach), tea (2.3–5.1 μg/g) and herbs and spices (71.0–123.6 μg/g). Intermediate manganese concentrations (range of 0.5–1.0 μg/g) were reported for cheese (0.05–1.0 μg/g), peppers (0.6–0.8 μg/g), grapes (0.5–0.8 μg/g) and sausages (0.4–1.0 μg/g). Lower manganese concentrations (range of 0.02–0.5 μg/g) were reported for meats (0.02–0.2 μg/g for beef, lamb, chicken, fishes, and veal), mushrooms (0.4–0.6 μg/g), apples (0.2–0.3 μg/g), coffee (0.2–0.3 μg/g), eggs (0.3–0.4 μg/g) and milk (0.01–0.04 μg/g).
The dietary intakes of different constituents for different age–sex groups of the Canadian population were also measured in the TDS (Health Canada, 2009). Manganese average dietary intakes were estimated in the 0–1, 2–3, 4–6, 7–9, 10–12 months old age groupings and for those 1–4, 5–11, 12–19, 20–39, 40–64 and 65+ years old. The average across all age groups of dietary intakes of manganese were estimated at 61.3 μg/kg body weight (bw) per day in 1993 to 1999 (Montreal), 59.0 μg/kg bw per day in 2000 (Ottawa), 51 μg/kg bw per day in 2001 (St John’s), 56.0 μg/kg bw per day in 2002 (Vancouver), 52 μg/kg bw per day in 2003 (Montreal), 50 μg/kg bw per day in 2004 (Winnipeg), 44 μg/kg bw per day in 2005 (Toronto), 53 μg/kg bw per day in 2006 (Halifax) and 54.0 μg/kg bw per day in 2007 (Vancouver). These manganese intakes support previous estimates of 2–9 mg/day for adults (higher levels for vegetarians) (Schroeder et al., 1966; Barceloux, 1999; IOM, 2001).
Average breast milk concentrations have been estimated at 3.7 μg/L (2.7–5.4 μg/L; n = 11 healthy mothers) during the first month, with the highest levels measured at day 1 (Casey et al., 1985). Levels decreased to 1.9 μg/L at 3 months, post-partum (Casey et al., 1985; IOM, 2001). Based on preliminary data, Health Canada has estimated a median content of 2.2 ng Mn/g in breast milk using the TDS measures (Health Canada, 2014b). Using this estimated median manganese level and assuming an average breast milk intake of 780 mL/day, dietary intake of manganese from breast milk for 0–6 month old infants can be calculated as ~ 0.2 µg/L (Health Canada, 2014b). The WHO has estimated a daily intake of manganese of 2.6–11.1 μg/day for breast fed infants (WHO, 2011).
In the U.S., daily intake from food was estimated at 2.7 and 2.2 mg for men and women, respectively (NRC, 1989). The intakes were estimated at 1.1 mg/day for infants aged 6–11 months, and at 1.5 mg/day for 2-year-old children. In the UK, average adult intake was estimated at 4.9 mg/day, with tea contributing to half of this amount (EVM, 2002).
With regards to manganese in infant formula, a minimum of 5 μg of manganese/100 Kcal (3.33 μg/100 mL of ready-to-feed infant formula) is indicated by the Canadian Food and Drug Regulations (Government of Canada, 2014). The regulations did not set a maximum for manganese in infant formula. The Expert Panel of the Life Science Research Office (LSRO) and the Codex Committee of the WHO and FAO have set guidelines at a minimum of 1 μg manganese/100 kcal and a maximum (guidance upper level (GUL) by WHO and FAO) of 100 μg manganese/100 Kcal (67 μg/100 mL) infant formula (liquid or powdered form) intended to be marketed as substitutes for breast milk in order to comply with nutritional requirements (Raiten et al., 1998; WHO, 2007).
5.3 Air
Ambient air generally has low levels of manganese, consistent with the low vapour pressure of the manganese compounds (Stokes et al., 1988). Low levels have also been measured in particulate matter (PM) in Canada. In Canada, ambient air manganese is monitored in atmospheric aerosols (fine/coarse) through the National Air Pollution Surveillance (NAPS) Network. In 2013, levels of manganese were measured in the range 0.050–51.4 ng/m3 for PM2.5 (vast majority of samples < 10 ng/m3) and 1–43 ng/m3 (0.001–0.043 μg/m3) for PM10 (vast majority of samples < 0.010 μg/m3) (Environment Canada, 2014). This is similar to what had been measured between 2003 and 2005, with manganese levels in the range of 0.002–0.025 μg/m3 (Health Canada 2010). The levels were between 0.06 and 0.22 μg/m3 in some areas of cities with major manganese-emitting industries (2003 and 2005). Levels of manganese (PM2.5 and PM10) have dropped between the late 1980s and early 2000s by 13–77% (Health Canada, 2010).
Studies investigating population exposure in Canadian and U.S. cities (Toronto, Riverside, and Indianapolis) were also conducted in Toronto in 1995 and 1996, years of widespread use of MMT in gasoline (Wallace and Slonecker, 1997). A median of 0.015 μg/m3 and 0.024 μg/m3 were measured for personal (general population) and fixed monitoring sites, respectively (limit of detection 2–9 ng/m3). In the United States, median of personal exposure was measured at 0.003 μg/m3 in Indianapolis (where MMT was not added to gasoline) and at 0.020–0.049 μg/m3 in Riverside, California. Levels of 1–2 ng/m3 were estimated as background concentrations in non-contaminated environments in Canada and the U.S.
5.4 Consumer products
Manganese is available as a nutritional supplement in Canada (Health Canada, 2007a). The Health Canada recommended doses in natural health products are between 0.13 and 9 mg/day for adults (none for less than 19 years old) from multiple sources including manganese chloride, citrate and oxide.
5.5 Soil
Manganese is naturally found in soil in concentrations estimated between 40 and 900 mg/kg. Depending on rock type, it can be found in concentrations outside of this range (higher in iron ores, mafic and sedimentary rocks, lower in sandstones) (Barceloux, 1999; IPCS, 1999; Michaelke et al., 2007).Children can ingest manganese in soil through hand-to-mouth behavior (IPCS, 1999).
The mean background (not influenced by anthropogenic activity) manganese concentration for different soil profiles of five major geological areas (n = 173) of Canada was 520 mg/kg, with a range of 100 to 1200 mg/kg in a comprehensive survey at the national and regional levels (McKeague and Wolynetz, 1980).
5.6 Biomarker concentrations in the Canadian population
Statistics Canada, Health Canada and the Public Health Agency of Canada launched Cycle 1 of the cross-sectional Canadian Health Measures Survey to collect health data and biological specimens in approximately 5600 Canadians aged 6–79 years distributed among five age groups (6–11, 12–19, 20–39, 40–59 and 60–79 years) at 15 sites between 2007 and 2009 (Health Canada, 2010). Cycle 2 of the Survey, conducted between 2009 and 2011 with a similar protocol, included Canadians aged 3–79 years old. For all ages, the geometric mean whole blood manganese concentration was 9.2 μg/L (95% confidence interval [CI] = 9.0–9.5 μg/L; n = 5309) in Cycle 1 and 9.8 μg/L (95% CI = 9.5–10.0 μg/L; n = 5575) in Cycle 2. Mean whole blood manganese concentration was in 11.0 μg/L (95% CI = 11.0–12.0 μg/L) in 3–5 year olds (cycle 1 only; cycle 2 not reported). Age groups older than 6 years were ranged from 8.8–10 μg/L in cycle 1 and 9.4–11 μg/L in cycle 2. Mean whole blood manganese concentrations from all age groups ranged from 8.6–9.5 μg/L in cycle 1, and 9–10 μg/L in cycle 2 for males, and 9.2–11 μg/L in cycle 1, and 9.5–11 μg/L in cycle 2 for females. The highest blood manganese levels were reported in the 6–11 years of age groups (10–12µg/L). The geometric mean urinary manganese concentration was 0.081 μg/L (95% CI = 0.072–0.092 μg/L; n = 5431), for the total Canadian population aged 6–79 years in Cycle 1 (not reported for Cycle 2 since most measurements were under the limit of detection). Whole blood manganese concentrations ranged from 6.3 μg/L (10th percentile) to 15.0 μg/L (95th percentile). No data were provided for children less than 3 years of age.
The Health Canada MIREC study (n = 1415–1938) reported geometric means in first trimester (8.8 μg/L 95% CI = 8.7–8.9 μg/L, maximum of 29.1 μg/L) and in third trimester of mother’s blood (12.0 μg/L 95% CI = 12.0–12.4 μg/L, maximum of 33.5 μg/L), in cord blood (30.9 μg/L 95% CI = 30.9–32.0 μg/L, maximum of 98.9 μg/L), and in meconium (4.5 μg/L 95% CI = 4.3–4.7 μg/L, maximum of 40.0 μg/L) (Health Canada, 2015).
Manganese geometric means of 20.4 μg/L, 0.36 μg/g, and 0.10 μg/g (5th and 95th percentiles of 11.1–40.4, 0.16–0.87, and 0.06–0.16 μg/L) were measured in mother’s blood, hair, and placenta, respectively, in a French cohort study (n = 247 pregnant women and their offspring) (Takser et al., 2003). Manganese geometric means of 38.5 and 0.75 μg/L (5th and 95th percentiles of 19.1–71.2, 0.22–4.25 μg/L) were measured in cord and newborn blood, respectively. Levels in blood at follow-up at 6 years old were very similar to the levels at birth.
In Quebec, a cross-sectional survey collected data on trace metals in men and women 18–65 years of age residing in the Quebec city area in 2001 (n = 500) (Leblanc et al., 2004). Levels of manganese in whole blood (geometric mean 169.8 nmol/L (95% CI = 164.9–174.8 nmol/L); n = 427) and serum (geometric mean 12.0 nmol/L (95% CI = 11.8–12.3 nmol/L); n = 403) were reported. Another study in Southwest Quebec looked at manganese exposure including blood levels (n = 306, aged 20–69 years old) (Baldwin et al., 1999). The geometric mean blood manganese was 7.1 μg/L (range 2.5–15.9). The geometric mean whole blood manganese concentration in a sample of non-smoking oyster growers (age 33–64 years old; n = 61) living in British Columbia was 176.8 nmol/L for males and 217.8 nmol/L for females, and 16.5 nmol/L for males and 12.9 nmol/L for females in serum (Clark et al., 2007). Although the blood manganese concentrations were significantly higher in females compared to males, serum manganese levels were not different between genders.
5.7 Total daily intake/exposure
Estimated intakes from drinking water can vary considerably depending on water manganese concentrations (see section 5.1 on exposure), and an accurate estimation of the exposure of the Canadian population is dependent on multiple factors. In addition, caution is merited when extrapolating the estimated intakes from various sources to the relative uptake from each source, as additional factors (including bioavailability and manganese form/speciation) play a role in the toxicological significance of the estimated dose.
5.8 Multi-route exposure through drinking water
Based on the physical and chemical properties of elemental manganese, exposure from drinking water through the dermal and inhalation routes is likely negligible (Karim, 2011).
6.0 Analytical methods
The U.S. Environmental Protection Agency (EPA) currently has four recommended analytical methods (Method 200.5 revision 4.2, Method 200.7. revision 4.4, Method 200.8 revision 5.4 and Method 200.9 revision 2.2) for the analysis of total manganese in drinking water (U.S. EPA, 2014). The EPA also recommends several methods (SM 3111B, SM 3113B, and SM 3120B) developed by a voluntary consensus standard organization.
Total manganese is defined as the sum concentration of both the dissolved and particulate (suspended) fractions of a water sample and is analyzed using methods to determine total recoverable manganese. Analysis of total manganese is needed for comparison to the MAC. However, determining the concentration of both the dissolved and particulate fractions is critical for determining the appropriate treatment method and monitoring treatment performance for manganese removal. This is discussed in more detail in sections 6.2 and 7.2.
The method detection limit (MDL) for each of the recommended methods is provided below. It should be noted, however, that MDLs are dependent on the sample matrix, instrumentation and selected operating conditions, and will vary between individual laboratories.
- EPA Method 200.5 Rev. 4.2 uses axially viewed inductively coupled plasma atomic emission spectrometry (ICP-AES) and has an MDL of 0.06 µg/L (U.S. EPA, 2003).
- EPA Method 200.7 Rev.4.4 uses ICP-AES and has an MDL of 1.4 µg/L (U.S. EPA, 1994a).
- EPA Method 200.8 Rev. 5.4 uses inductively coupled plasma mass spectrometry (ICP-MS) and has MDLs of 0.02 µg/L for total recoverable manganese and 0.04 µg/L for direct aqueous injection (U.S. EPA, 1994b).
- EPA Method 200.9 Rev. 2.2 uses stabilized temperature graphite furnace atomic absorption (GFAA) spectrometry and has an MDL of 0.3 µg/L (U.S. EPA, 1994c).
- SM 3111B uses direct air-acetylene atomic absorption spectrometry and has an MDL of 0.01 mg/L (10 µg/L) (APHA et al., 1992, 1995, 2005, 2012).
- SM 3113B uses electrothermal atomic absorption spectrometry and has an MDL of 0.2 µg/L (APHA et al., 1992, 1995, 2005, 2012).
- SM 3120B uses ICP-AES and has an MDL of 2 µg/L (APHA et al., 1992, 1995, 1998, 2005, 2012).
- The online versions of SM 3113B-04, 99 and SM 3120B-99 are also recommended methods.
One additional standardized analytical method is available that is not included in the U.S. EPA recommended methods. SM3125 is a well-established method that is acceptable for conducting manganese analyses in drinking water.
- SM 3125B uses ICP-MS and has an MDL of 0.002 µg/L (APHA et al., 1992, 1995, 2005, 2012).
6.1 Methods
EPA Method 200.5 revision 4.2, EPA Method 200.7 revision 4.4 and SM3120B are based on multi-elemental determinations by inductively coupled plasma atomic emission signals (ICP-AES) using sequential or simultaneous instruments. The instruments measure characteristic atomic-line emission spectra by optical spectrometry. Element specific emission spectra are produced by radio-frequency inductively coupled plasma (ICP). The spectra are dispersed by a grating spectrometer and the intensities of the line spectra are monitored at specific wavelengths by a photosensitive device. A variation for EPA Method 200.5 is that it uses axially viewed plasma atomic emission signals for the analysis of all analytes. Matrix effects may occur in EPA 200.5 when total concentrations of calcium, magnesium, and sodium exceed 125 mg/L and silica exceeds 250 mg/L. Interferences may occur in EPA Method 200.7 and SM3120B when total dissolved solids are greater than 0.2% (w/v) or 1500 mg/L, respectively.
In EPA Method 200.8 Rev. 5.4 and SM 3125 B, samples are atomized and ionized into a radio-frequency plasma. The ions are extracted from the plasma by a vacuum interface and separated on the basis of their mass-to-charge ratio by a mass spectrometer. Separated ions are detected by an electron multiplier or Faraday detector. Matrix effects may occur in EPA Method 200.8 and SM 3125B when total dissolved solids are greater than 0.2% and 0.5% (w/v), respectively.
Method 200.9 Rev. 2.2 and SM 3113B use stabilized temperature platform graphite furnace atomic absorption spectrometry. The technique includes a series of three heating steps to dry, char (to reduce interferences by other ions) and atomize analytes from the pyrolytic graphite surface. The atomization raises the analytes into an atmosphere of high-purity argon, and light of a specific wavelength is passed through the atomic cloud. The attenuation of the intensity of light is measured. No matrix interferences relevant to manganese were identified in these methods.
SM 3111B is a direct air-acetylene flame atomic absorption method. The sample is aspirated into the flame and atomized. A light beam is directed through the flame, into a monochromator, and onto a detector that measures the amount of light absorbed by the atomized element in the flame. Because each metal has its own characteristic absorption wavelength, a source lamp composed of that element is used.
There is no U.S. EPA practical quantitation level (PQL) for manganese. However, a U.S. EPA (2010) report indicated that the use of PQLs for determining analytical capabilities can be problematic as different methods have been used for its determination. The report noted that minimum reporting levels (MRLs) may be useful as an alternative to PQLs. The MRL for an analyte is measured using a specific analytical method and is defined as an estimate of the lowest concentration minimum reporting level or LCMRL that is achievable by the analyst with 95% confidence at least 75% of the time (U.S. EPA, 2010). MRLs were not reported for the methods listed above with the exception of EPA Method 200.5 that reported an MRL of 0.2 µg/L. With the exception of EPA Method 3111B, the reported MRLs and/or MDLs of the methods listed above are well below the levels needed for accurate quantitation of manganese below the MAC and AO.
6.2 Sample preservation and preparation
Accurate quantification of dissolved, particulate and total manganese in samples is dependent on the proper sample preservation and preparation steps. SM 3030B provides guidance on filtration and preservation procedures for the determination of dissolved or particulate metals (APHA et al., 2012). To determine dissolved metals, samples should be filtered at the time of collection using preconditioned plastic filtering devices under either vacuum or pressure. Membrane filters with pore diameter sizes between 0.22 to 0.45 µm are recommended for fractionating dissolved and particulate manganese (Kohl and Medlar, 2006; Brandhuber et al., 2013). The filtrate should be acidified to pH < 2 with concentrated nitric acid. To determine particulate manganese, the filter should be retained and the particulate material on it digested in the laboratory using appropriate methods (APHA et al., 2012).
Currently, EPA methods 200.7, 200.8 and 200.9 and SM 3111B, SM 3113B, SM 3120B and SM 3125B require acid digestion of samples only when the turbidity of the acid-preserved sample is greater than one (1) nephelometric turbidity unit (NTU). Digestion for EPA methods is performed by transferring an aliquot of the sample into a solution of nitric acid and hydrochloric acid followed by gentle heating to a temperature of approximately 85°C (i.e., hot digestion) (EPA, 1994,a,b,c). Microwave-assisted digestion (SM 3030 K) is recommended for analysis of total recoverable manganese using SM methods that are based on ICP-MS. Although the methods cited above do not require hot acid digestion for total manganese analysis unless turbidity is greater than 1 NTU, research conducted on other metals such as lead and chromium has indicated that this does not accurately quantify the total metal concentration in a sample. For example, analytical requirements under UCMR3 include solubilizing the acid-preserved sample by gentle heating using nitric acid, regardless of the sample turbidity or the method used (U.S. EPA, 2012). Similarly, APHA et al. (2012) recommends verifying if adequate recovery of metals has occurred in different sample matrices by comparing digested and undigested results.
In some cases, utilities may want to quantify the fraction of colloidal manganese present within a treatment plant or in the finished water. This could be of particular importance when troubleshooting problems with treatment plant operations. Colloidal manganese can be found in water samples collected following the application of a strong oxidant such as ozone, permanganate or chlorine dioxide, prior to coagulation, or in treatment plants where intermediate ozonation is practiced (i.e., biological filtration plants). These colloidal solids are also more likely to be found in soft waters as calcium and magnesium ions help to destabilise and aggregate manganese oxide particles. Colloidal manganese is typically defined as manganese oxide particles that are < 0.20 µm and > 30,000 daltons. If colloidal manganese is present it will pass through 0.20 µm filters and it will be incorrectly quantified as dissolved manganese. Therefore, following initial filtering through a 0.20 µm filter, the sample will require additional filtering through a 30,000 molecular weight cut-off (MWCO) filter when quantification of colloidal manganese is needed.
6.3 Colorimetric methods
Colorimetric methods are available for quantifying dissolved manganese in drinking water. These methods are based on a reaction between dissolved manganese and other compounds to produce permanganate which can then be measured colorimetrically. These reactions can be subject to interferences from other metals or organics in solution which can affect the accuracy of the results. Therefore, these methods are best suited to monitoring within treatment plants to assess treatment effectiveness and not for compliance monitoring. The advantage of colorimetric methods is that commercial kits are available and they can be used to obtain a rapid indication of changes to manganese concentrations, which can aid process monitoring within a treatment plant (Brandhuber et al., 2013).
SM 3500 Mn B is a colorimetric methods based on persulfate oxidation of dissolved manganese in the presence of silver nitrate. Samples with particulate manganese oxides need to be treated with a series of reducing compounds to dissolve the manganese oxides. Photometric measurements can be made by preparing standard solutions and comparing the sample absorbance to those of the standards. This method has a minimum detectable concentration of 42 µg/L (APHA et al., 2012). A proprietary colorimetric method that employs ascorbic acid, alkaline cyanide and an indicator solution is also available. This method has a detection range of 0.01 to 0.7 mg/L (Brandhuber et al., 2013).
7.0 Treatment technology and distribution system considerations
7.1 Manganese chemistry and treatment
An understanding of the chemistry and microbiology of manganese in drinking water systems is important when determining the most appropriate treatment system or assessing and optimizing existing systems for manganese removal. As discussed in section 4.0, manganese exists in many oxidation states in water systems. The four most important oxidation states from a drinking water treatment perspective are Mn(II), a soluble free metal divalent cation that is clear in water, Mn(III), a transitional state which exists as a manganese oxide (Mn2O3) that is a dark brown/black solid, Mn(IV) as MnO2(s), a black solid, and Mn (VII) as MnO4–, a dissolved permanganate ion that appears purple in water and is added to water as an oxidant during drinking water treatment. A brief overview of manganese chemistry relevant to drinking water treatment is provided below. More detailed information can be found in Sommerfield (1999), Kohl and Medlar (2006), and Brandhuber et al. (2013).
The chemical reactions that control which species of manganese are present in drinking water treatment include oxidation/reduction, precipitation/dissolution, and sorption/desorption reactions. In a drinking water treatment plant, all three reactions can occur throughout the treatment process. The species of manganese which are present are controlled by the oxidation/reduction potential and pH of the water along with the presence of other parameters in the water that can combine with manganese to form manganese compounds. Under high pH or strongly oxidizing conditions, dissolved Mn(II) will, at equilibrium, form insoluble oxides, hydroxides or carbonate solids.
Manganese treatment processes are designed to take advantage of the differences in the solubility of manganese species. The solubility of reduced Mn(II) is orders of magnitude greater than the solubility of Mn(IV). Therefore, oxidation of Mn(II) to Mn(IV) results in precipitation of manganese oxide solids. Since these solids can exist in several oxidation states (e.g., Mn(III) and Mn(IV)) they are often referred to as MnOx(s) (Brandhuber et al., 2013). The tendency of manganese to precipitate or dissolve can be controlled by changing the oxidation/reduction and pH conditions in a drinking water treatment plant.
Adsorption/desorption is the third process that controls manganese removal in drinking water treatment plants. MnOx(s) particulates have an electric charge that varies with pH, but in most cases, these particles are negatively charged and have the capability to adsorb Mn(II) ions from solution. Once Mn(II) is adsorbed to MnOx(s) particles, the surface catalyzes oxidation of the adsorbed Mn(II) to Mn(IV). This increases the rate at which Mn(II) can be oxidized to Mn(IV).
In practice, manganese can convert between the soluble and particulate forms and can be challenging to control to low levels (i.e., < 0.015 mg/L) in treatment plants and distribution systems (Brandhuber et al., 2013). It is also important to note that MnOx(s) can exist in both particulate and colloidal forms. The particulate form is comprised of large oxidized solids (> 0.20–0.45 µm) whereas the colloidal form is comprised of smaller oxidized particles (< 0.20 µm and > 30,000 daltons). Under certain conditions, substantial amounts of colloidal manganese can be formed during the direct oxidation of Mn(II) with a strong oxidant such as ozone. Colloidal particles can be difficult to destabilize and coagulant addition needs to be optimized to effectively remove these particles during sedimentation and filtration (Casale et al., 2002; Brandhuber et al., 2013).
7.2 Municipal scale treatment
In order to select the appropriate treatment system for manganese removal or optimize an existing system, it is critical to have a good understanding of the form of manganese (dissolved, colloidal or particulate) present in the source water. When source water needs to be treated for manganese removal, most often dissolved Mn(II) is the predominant species present (i.e., in anoxic groundwater or lake). However, depending on the pH and the dissolved oxygen (DO) content of the water, a combination of dissolved and particulate manganese can be present. In general, treatment methods used for manganese rely on a combination of processes to remove both dissolved and particulate forms of manganese (e.g., oxidation, adsorption and/or filtration). If manganese is present only in colloidal or particulate form (Mn(IV)), it can be removed using common particle removal processes such as conventional filtration or low pressure membrane filtration (microfiltration or ultrafiltration).
Dissolved manganese as Mn(II) can be removed or controlled by source water practices, oxidation/physical separation, adsorption/oxidation, biological filtration, and precipitative softening. One of the most commonly used technologies effective for decreasing manganese concentrations in drinking water is based on directly oxidizing dissolved Mn(II) to form particulate MnOx(s), which are then removed by a physical removal process such as clarification and granular media filtration or low pressure membrane filtration. These processes typically remove 80 to 99% of manganese to achieve treated water concentrations below 0.04 mg/L. Another common treatment technique for manganese removal is the use of MnOx(s) coated filter media that adsorbs dissolved Mn(II) and then oxidizes it at the surface when coupled with the presence of an appropriate oxidant such as free chlorine or potassium permanganate. This treatment technology generally achieves higher levels of manganese removal to achieve treated water concentrations below 0.020 mg/L. Biological filtration and softening have also been found to be effective for manganese removal achieving treated water concentrations below 0.03 mg/L (Casale et al., 2002; Kohl and Medlar, 2006; Tobiason et al., 2008; Kohl and Dixon, 2012; Brandhuber et al., 2013). Many of these technologies, in particular MnOx(s) coated filter media, can be used to effectively remove manganese from drinking water in small systems. Utilities may be able to achieve lower treated water manganese concentrations than those indicated above depending on site-specific design and operation of the treatment plant. In particular, optimization of existing treatment plants has been shown to effectively lower manganese concentrations below 0.02 mg/L (Brandhuber et al., 2013).
The most effective type of treatment for manganese removal will depend on the type and concentration of manganese in the source water, overall water chemistry, treatment process selected, and other water quality objectives. Utilities should conduct monitoring within treatment plants, including periodically determining the different forms of manganese (e.g., dissolved and particulate) to ensure that unit processes are adequately removing manganese. Extensive guidance on the treatment of manganese in drinking water systems can be found in Brandhuber et al. (2013).
Historically, limits for manganese in treated water have been based on aesthetic considerations and treatment achievability (U.S. EPA, 1979). More recently, studies have shown that MnOx(s) particulates in drinking water cause discolouration of water and are visible to consumers at concentrations as low as 0.005–0.02 mg/L (Sly et al., 1990; Sain et al., 2014). In addition, extensive surveys have found that a manganese concentration of 0.05 mg/L in treated water is not sufficiently low to ensure minimal consumer complaints (Sly et al., 1990; Casale et al., 2002; Kohl and Medlar, 2006). Based on detailed utility surveys, Kohl and Medlar (2006) reported that the majority of consumers experience episodic difficulties with water discolouration when manganese concentrations are 0.05 mg/L at the tap. Many studies have found that existing treatment plants are capable of achieving a treated water manganese concentration of less than 0.02 mg/L through optimized design and operation of new or existing treatment plants (Kohl and Medlar, 2006; Tobiason et al., 2008; Brandhuber et al., 2013). Kohl and Medlar (2006) reported data from a survey of 189 treatment plants removing manganese from groundwater and surface water sources using a variety of treatment technologies. The geometric mean of the average treated water concentrations from these plants was 0.010 mg/L. Similarly, Casale et al. (2002) reported that approximately 80% of groundwater and 70% of surface water treatment plants (193 treatment plants) achieved treated water concentrations of 0.01 mg/L. These data indicate that utilities can achieve lower treated water concentrations than previously reported and that a manganese concentration below 0.02 mg/L in treated water is achievable for most utilities (Casale et al., 2002; Kohl and Medlar; 2006; Tobiason et al., 2008; Brandhuber et al., 2013).
The implications of the accumulation and release of manganese and co-occurring metals in distribution systems has also been the subject of extensive research in recent years (U.S. EPA, 2006; Friedman et al., 2010; Ginige et al., 2011; Brandhuber et al., 2015). Elevated manganese concentrations at the tap can be associated with the accumulation and release of manganese in the distribution system rather than elevated manganese in the source or treated water (Brandhuber et al., 2015).
Based on the information discussed above, it is recommended that treatment plants establish a treated water goal of ≤ 0.015 mg/L of total manganese (Brandhuber et al., 2013), which is achievable in most well operated and optimized treatment plants. As the establishment of such a goal will minimize the accumulation of manganese in the distribution system, it will also minimize the subsequent release of manganese in the distributed water, preventing both consumer complaints related to discoloured water and higher manganese concentrations at consumers’ taps (which could be above the MAC).
7.2.1 Source water control
In some cases, it may be possible to lower the levels of manganese entering a treatment plant by managing the levels occurring in the source water. Manganese concentrations in groundwater from an individual well tend to be less variable temporally (daily or seasonally) than in surface water (Barbeau et al., 2011). However, large variations in manganese concentrations have been observed between different wells in the same well field located in close proximity. Therefore, utilities with multiple wells can selectively pump different combinations of wells at different flow rates to achieve a lower overall manganese concentration in the water entering the treatment plant (Brandhuber et al., 2013). Another option for controlling manganese concentrations in groundwater is to oxidize dissolved Mn(II) in situ by raising the redox potential in the aquifer surrounding the wells. This can be achieved by injecting aerated water into the aquifer through recharge wells. This technology is not often used due to concerns with altering the aquifer geochemistry and risk of clogging the extraction wells (Mettler et al., 2001). Despite these concerns, full-scale applications of this technology have been operating successfully in Switzerland and the United States (AWWA, 1984; Mettler et al., 2001).
Aeration techniques can also be used as a control option for lowering manganese levels in surface water. Reservoirs and lakes can experience a complete loss of dissolved oxygen (DO) in the hypolimnion during thermal stratification. This can result in an increase in the concentration of dissolved Mn(II) in the water column due to release from sediments under anoxic conditions. Hypolimnetic aeration and oxygenation can be used to add DO to reservoirs while maintaining stratification (Brandhuber et al., 2013). There are several types of aerators that can be used that generate a stream of gas in contact with water to transfer oxygen to the water which is then delivered to the hypolimnion. The amount of oxygen required to adequately aerate a portion or all of the hypolimnion depends on the volume of water, the DO profile in the lake and the oxygen demand of the sediments. Careful control is needed to avoid reservoir destratification, which can lead to other water quality issues (Kohl and Medlar, 2006). Brandhuber et al. (2013) reported data from a case study of a full-scale hypolimnetic aeration system installed in a reservoir experiencing thermal stratification and resulting in Mn(II) concentrations of 1.7 mg/L at the plant intake. After considering a number of treatment options, the utility decided to install a liquid oxygen system that consisted of two gaseous oxygen diffuser lines placed at the bottom of the reservoir. The system was operated from April to October with oxygen flows adjusted to maintain DO concentrations above 5 mg/L. This minimized the occurrence of dissolved Mn(II) released from the sediment and lowered source water concentrations to a year-round average below 0.05 mg/L (maximum value below 0.2 mg/L).
Variable depth intake is also a control option for surface water treatment plants that have deep reservoirs and a multilevel intake system. These systems can select the level in the reservoir from which to draw water into a plant based on the water quality present at different depths. When minimizing manganese concentrations is the primary objective, treatment plants can monitor the DO and dissolved Mn(II) concentration at each intake and take water with the lowest concentrations (typically from shallower depths once the hypolimnion becomes anoxic in a reservoir). In practice, a variety of water quality considerations are typically taken into consideration when selecting the depth of intake to use since drawing water from shallower depths can lead to other water quality issues, such as increased algal cell counts or taste and odour problems (Benskin et al., 2004; Brandhuber et al., 2013).
7.2.2 Chemical oxidation / physical separation
When the predominant form of manganese in the source water is dissolved Mn(II), then direct oxidation to convert Mn(II) to MnOx(s) precipitates followed by physical separation can be effective for manganese removal from drinking water. The effectiveness of these processes for manganese removal depends largely on the ability of the oxidant(s) to completely convert manganese from its dissolved state Mn(II) to solid MnOx(s). Effective oxidation of manganese depends on several factors, including pH and Eh, temperature, reaction time, alkalinity, and the total oxidant demand in the water (e.g., presence of iron, sulphide, nitrate, ammonia and organic compounds) (Casale et al., 2002; Brandhuber et al., 2013). Once dissolved Mn(II) has been converted to particulate MnOx(s), removal can be achieved using coagulation/flocculation, sedimentation or dissolved air flotation and granular media filtration. Low pressure membrane filtration (ultra and microfiltration) can also be used. The effectiveness of physical removal processes is dependent on ensuring that the manganese entering the filter is in the particulate form (Tobiason et al., 2008). In addition, Brandhuber et al. (2013) indicated that a key aspect of effective removal of manganese particles depends on their size and on the location in the treatment train in which they are generated. For example, colloidal particles need to be destabilized using coagulation/flocculation prior to filtration.
Oxidation of manganese in water is an autocatalytic three-step reaction process with different reaction kinetics for each step, which has important implications for drinking water treatment. The first step is solution-phase oxidation of Mn(II) by the oxidant (relatively slow step), the second step is adsorption of Mn(II) to MnOx(s) solids (fast step) and the third step is the surface-catalyzed oxidation of the sorbed Mn(II) ion by additional oxidant (moderate step). It should be noted that the second step is important in filtration processes as well because MnOx(s) coatings present on filter media rapidly adsorb dissolved Mn(II) which can then be oxidized. This is discussed in greater detail in section 7.2.3.
The chemical oxidants typically used to convert dissolved Mn(II) to MnOx(s) precipitates include permanganate (MnO4–), chlorine dioxide (ClO2) and ozone (O3). Under high pH conditions, chlorine and oxygen may also be effective. Studies conducted by Knocke et al. (1990a) and Gregory and Carlson (2003) indicated that the kinetics of the reactions of these oxidants with Mn(II) are sufficiently rapid under most pH, temperature, dissolved organic carbon (DOC) and initial Mn(II) concentrations to be used in drinking water treatment processes. Oxidation using chlorine and oxygen is not effective unless pH is greater than 9 or 9.5, respectively (Knocke et al., 1990a; Brandhuber et al., 2013). Detailed information on the effectiveness of different oxidants for manganese removal in drinking water is available in Knocke et al. (1990a), Casale et al. (2002), Gregory and Carlson (2003), Kohl and Medlar (2006), Tobiason et al. (2008) and Brandhuber et al. (2013).
Brandhuber et al. (2013) reported on the key factors that affect the efficiency of the chemical oxidation of Mn(II), including oxidant selection, dosing, reaction kinetics, reaction time location of oxidant addition, and monitoring. The most important operational factor is to allow sufficient reaction time in the treatment plant for complete and efficient Mn(II) oxidation. Typically for reaction rates to be sufficiently rapid for effective oxidation in a treatment plant, addition of a strong oxidant at an appropriate pH is needed (Brandhuber et al., 2013). In addition, oxidant doses greater than the stoichiometric ratios are needed to meet the oxidant demand of the source water and achieve adequate oxidation of manganese (Kohl and Medlar, 2006). In particular, the presence of iron, which often co-occurs with manganese in source water, can impact the efficiency of manganese oxidation. Iron is easier to oxidize than manganese at any pH, so the oxidant demand from iron must be satisfied before significant manganese oxidation will occur (Brandhuber et al., 2013). Utilities should conduct jar testing to assess which oxidants may be effective with their source water and treatment plant design prior to selecting an oxidant.
When using permanganate, chlorine dioxide and ozone to oxidize Mn(II), colloidal MnOx(s) with particles sizes below one (1) µm can be created (Knocke et al., 1990a; Brandhuber et al., 2013). This colloidal MnOx(s) formation is seen more often in softer, low hardness waters as the presence of dissolved calcium and magnesium ions helps to destabilize and aggregate these colloids. It is generally recommended that these oxidants be added prior to coagulation/flocculation processes so that the colloidal particles can be destabilized and removed through conventional sedimentation and filtration processes (Brandhuber et al., 2013).
Many different combinations of oxidation and physical separation are used for manganese treatment, including the use of multiple oxidants, variations in placement of the oxidant addition, and different physical separation methods. Considerations for each oxidant and filtration technology are discussed in the following sections. The capabilities of these technologies for removal of dissolved manganese with examples of full- and pilot-scale treatment plants are also reported below, recognizing that the effectiveness of any treatment process is site-specific and varies based on source water quality and the design and operation of each plant.
7.2.2.1 Permanganate
Oxidation of dissolved Mn(II) can be achieved using permanganate (MnO4–) supplied in either the sodium (NaMnO4) or potassium (KMnO4) forms. Historically, KMnO4 was the predominant form of permanganate used for oxidation of Mn(II). However, Brandhuber et al. (2013) noted that an increasing number of utilities are using NaMnO4 because it can be purchased as a highly concentrated solution which eliminates the need for equipment at the facility for dissolution of the dry product.
Data reported on the oxidation of Mn(II) using permanganate indicate that it occurs rapidly under a wide range of temperature and pH conditions in water with low DOC (< 3 mg/L) (Knocke et al., 1987 and 1990). The stoichiometric dosage of permanganate required to oxidize Mn(II) to Mn(IV) is 1.9 mg KMnO4 per mg Mn(II); however, as with all oxidation reactions, the demand from other parameters found in the source water can increase the overall dosage required. Knocke et al. (1990a) demonstrated that for a pH range of 5.5 to 9.0, oxidation of 0.25 mg/L of Mn(II) by permanganate at 105% of the stoichiometric requirement occurred within 60 seconds (T = 25°C, DOC < 1 mg/L). Increasing the DOC to 10 mg/L decreased the rate of Mn(II) oxidation to 1–2 minutes (T = 25°C, pH = 7.0). Conditions in which oxidation was not effective at lowering final Mn(II) residuals to levels below 0.05 mg/L included a temperature of 5°C and a pH of 5.5. The authors noted that using permanganate to oxidize Mn(II) when significant organic matter is present in the source water may be impractical due to high chemical costs (Knocke et al., 1987). Work conducted by Gregory and Carlson (2003) found that reaction times for oxidation of Mn(II) with permanganate increased with lower initial Mn(II) concentrations. For an initial Mn(II) concentration of 60 µg/L and a 300% stoichiometric dose of KMnO4, 1,200 seconds were required to achieve a residual Mn(II) concentration below 20 µg/L (treated water goal for the study). In comparison, the oxidation rate at 1,000 µg/L was far more rapid and resulted in oxidation of Mn(II) to a concentration below 10 µg/L within less than 200 seconds. The water quality for these tests was pH =7.0, TOC = 3.4 mg/L and T = 9°C.
In practice, dosing of permanganate to oxidize Mn(II) must be precisely optimized to completely oxidize Mn(II) without resulting in any excess permanganate in the treated water. Excess permanganate exiting the drinking water treatment plant can result in pink water. It can also lead to MnOx(s) precipitation in the distribution resulting in an increase in consumer complaints due to discoloured water. Therefore, careful consideration to the contact time and the permanganate dose is needed in this process. Brandhuber et al. (2013) reported that for most treatment plants, a reaction time of 2 to 4 minutes is sufficient for lower initial Mn(II) concentrations and/or lower pH values (e.g., pH 6–6.5) but that shorter reaction times may often be possible for higher pH conditions.
Many studies have demonstrated the effective removal of dissolved Mn(II) in full-scale treatment plants using oxidation with permanganate followed by physical separation (Carlson et al.,1997; Kohl and Medlar, 2006; Brandhuber et al., 2013). Kohl and Medlar (2006) reported data from seven full-scale treatment plants using KMnO4 addition followed by filtration for manganese removal. In all cases, the plants were capable of achieving greater than 80% removal of manganese to achieve average treated water concentrations less than 0.045 mg/L. Two of the plants using oxidation with KMnO4, followed by conventional mixing, flocculation, settling and granular media filtration (conventional filtration), were able to achieve between 80% and 86% removal of manganese from average influent concentrations of 0.090 and 0.111 mg/L down to average treated water concentrations of 0.019 mg/L to 0.021 mg/L, respectively. Utilities that coupled KMnO4 with additional oxidation achieved higher manganese removals. Two plants using a combination of aeration, KMnO4 and conventional filtration removed 92 and 97% of manganese to achieve average treated water concentrations of 0.019 and 0.045 mg/L. Dissolved air flotation has also been used to remove oxidized manganese. An Australian utility combined pre-treatment with potassium permanganate, followed by polyaluminum chlorohydrate addition, flocculation and dissolved air flotation, to successfully remove manganese (Kohl and Medlar, 2006). The concentration of manganese was reduced from 0.08 mg/L in the raw water to less than 0.02 mg/L in the treated water. No operational data were available for the treatment plants discussed above.
Several case studies of techniques for optimizing systems using KMnO4 oxidation followed by conventional filtration have been reported in the literature (Brandhuber et al., 2013). A utility treating a lake water source with a highly variable Mn(II) concentration in the raw water (0.2 to 1.0 mg/L fluctuations hourly) was optimized using oxidation/reduction potential (ORP) measurements to control the permanganate feed in the plant. The plant changed from using flow-controlled chemical addition to using ORP controlled dosing. Using this method, treated water manganese concentration were below 0.01 mg/L 90% of the time and below 0.02 mg/L 95% of the time.
The combination of oxidation of Mn(II) using KMnO4 and low pressure membrane filtration for removal of manganese has also been applied in full-scale drinking water treatment plants. For facilities that use oxidant-resistant membranes, permanganate is the oxidant of choice. In these cases it is important that the oxidation reactions are completed before the water reaches the membranes, as formation of precipitates within the membrane pores can damage the membrane structure. A full-scale potassium permanganate/ultrafiltration plant successfully treated raw water with a manganese concentration of 0.62 mg/L and an iron concentration of 0.4 mg/L to achieve treated water concentrations below the detection limit (not provided) (AWWA, 2005). The plant operates at a net flux of 25 gfd and consists of KMnO4 addition followed by static mixing, an oxidation chamber, and three parallel immersed membrane tanks. A reclaim tank receives the reject water goes and the supernatant is recycled back to the head of the plant, resulting in an overall system recovery of 99%. Kohl and Medlar (2006) also reported high manganese removals for a full-scale drinking water treatment plant using KMnO4 followed by microfiltration. The plant reported achieving 99% removal of manganese from an average influent concentration of 0.811 mg/L down to 0.005 mg/L. Operational data were not available for this treatment plant (AWWA, 2005).
7.2.2.2 Chlorine dioxide
Chlorine dioxide can effectively oxidize dissolved Mn(II) to Mn(IV), although it is best suited for source waters that do not have a high oxidant demand from organic matter (Tobiason et al., 2008). Reaction rates between ClO2 and dissolved Mn(II) are rapid, with typical reaction times of 1–2 minutes or less, depending on the dosage of ClO2 and the initial dissolved Mn(II) concentration (Brandhuber et al., 2013). Similar to permanganate, a significant decrease in oxidant efficiency was observed at low temperatures (5°C) and low pH (5.5) (Knocke et al., 1990a). The stoichiometric dosage of ClO2 required for oxidation of Mn(II) is 2.45 mg ClO2 per mg Mn(II). However, in the presence of Mn(II), chlorine dioxide is not completely reduced to a chloride ion (Cl-); instead it is reduced only to chlorite (ClO2-) (Knocke et al., 1990a). In addition, it should be noted that if chlorine dioxide and chlorite ion are not removed prior to secondary disinfection with chlorine, they will react with free chlorine to form chlorate ion. Once chlorate ion is present in water, it is very persistent and very difficult to remove. In Canada, chlorite and chlorate have health-based drinking water guidelines of 1 mg/L. However, to ensure that the chlorite and chlorate guidelines can be met, it is recommended that treatment plants using chlorine dioxide as a primary disinfectant not exceed a feed dose of 1.2 mg/L (Health Canada, 2008).
Knocke et al. (1987) demonstrated that a dosage of 1–1.5 mg/L effectively oxidized initial Mn(II) concentrations of approximately 0.25 mg/L over a wide pH range when DOC was below 2.5 mg/L. In contrast, water with DOC between 8–10 mg/L required more than 3 mg/L of ClO2. Gregory and Carlson (2003) compared oxidation of Mn(II) with chlorine dioxide, potassium permanganate and ozone at lower initial Mn(II) concentrations (60 µg/L) and found that chlorine dioxide was the most effective for achieving treated water concentrations < 10 µg/L. At a relative stoichiometric ClO2 dose of 200%, oxidation of 60 µg/L down to less than 10 µg/L occurred within 300 seconds in water with T = 9°C, pH = 7.0, and TOC = 3.4 mg/L. This was reduced to less than 75 seconds when the initial Mn(II) concentration was 200 µg/L.
Given that chlorine dioxide dosing needs to be controlled to below 1.2 mg/L, several authors have noted that the application of only chlorine dioxide for Mn(II) oxidation is limited to waters with low DOC and relatively low dissolved Mn(II) levels that require oxidation for treatment. In some cases the addition of permanganate in conjunction with chlorine dioxide may be effective for higher DOC waters (Knocke et al., 1987; Casale et al., 2002; Brandhuber et al., 2013). An additional consideration is that on-site generation of chlorine dioxide is required due to its high degree of reactivity.
Despite these limitations, several full-scale treatment plants have reported using chlorine dioxide followed by physical separation for manganese removal. Kohl and Medlar (2006) reported data from several plants using chlorine dioxide followed by conventional filtration that were capable of 81–95% removal of manganese, to achieve average treated water concentrations as low as 0.001 mg/L. In a case study reported by Brandhuber et al. (2013), a full-scale treatment plant that was experiencing coloured water complaints successfully switched from using potassium permanganate to chlorine dioxide for oxidation of raw water concentrations of Mn(II) between 0.07 and 0.2 mg/L. The utility found that ClO2 was more effective at controlling Mn(II) concentrations to below 0.02 mg/L. Similarly, studies to determine the most effective oxidant for Mn(II) removal found that ClO2 was more effective than potassium permanganate and ozone for achieving low levels of Mn(II). Chlorine dioxide doses ranging from 0.44 to 1.5 mg/L effectively oxidized Mn(II) to 0.005 mg/L without creating unacceptable levels of chlorite (Carlson and Gregory, 2003).
The combination of oxidation using chlorine dioxide and microfiltration is also effective at removing manganese from drinking water. Tobiason et al. (2008) reported that a pilot-scale system using 0.5 mg/L of chlorine dioxide as the pre-filter oxidant and microfiltration was capable of reducing an influent manganese concentration of 0.094 mg/L down to 0.001 mg/L.
7.2.2.3 Ozone
Ozone is a strong oxidant that can be effective for Mn(II) oxidation, although it is considered less effective than other oxidants for achieving levels of manganese below 0.02 mg/L in drinking water (Brandhuber et al., 2013). Typically, oxidation using ozone in drinking water treatment processes occurs with molecular O3 and the uncharged hydroxyl radical OH. In the case of oxidation of dissolved Mn(II), studies have shown that it is molecular O3 that reacts directly with Mn(II) and hydroxyl radicals are relatively ineffective (Nowell and Hoigne, 1987). The stoichiometric requirement for Mn(II) oxidation is 0.87 mg O3 per mg Mn(II) (Knocke et al., 1990a). The actual dosage needed is usually 2 to 5 times higher depending on the alkalinity and DOC of the source water. The presence of DOC in source water significantly inhibits oxidation of Mn(II) by ozone due to the competitive oxidant demand exerted by the DOC (Knocke et al., 1990a). This effect can be minimized by increasing the alkalinity which promotes direct oxidation of Mn(II) by O3 due to less hydroxyl radical formation (Paillard et al., 1989). The kinetics of Mn(II) oxidation by ozone are similar to those observed with permanganate and chlorine dioxide under similar pH and temperature conditions, although the manganese residual levels tend to be higher. Knocke et al. (1990a) and Carlson and Gregory (2003) reported that oxidation of Mn(II) with ozone occurred rapidly (30–60 seconds) with various ozone doses (0.5–2.0 mg/L) for various pH (5.63–7.0), DOC (1 and 3.4 mg/L) and temperature (9 and 25°C) conditions but low levels of residual manganese (< 0.02 mg/L) were not observed in these experiments.
Several authors have noted that optimizing ozone dosing can be challenging due to competitive oxidant demands (Kohl and Medlar, 2006; Tobiason et al., 2008; Brandhuber et al., 2013). Ozone oxidizes not only dissolved Mn(II) but at high enough doses it can also oxidize Mn(III) and Mn(IV) oxide particles to the Mn(VII) oxidation state and produce permanganate, which can result in discolouration of the water (pink water). Gregory and Carlson (2003) noted that to achieve effective Mn(II) removal using ozone, the background demand exerted by NOM required the use of ozone dosages that were high enough to result in the production of Mn(VII) and discolouration of the treated water.
Limited pilot-scale studies have been conducted examining the use of ozonation followed by filtration for manganese removal (McKnight et al., 1993; Wilczak et al., 1993). McKnight et al. (1993) conducted pilot-scale testing to determine if ozone could be used to replace chlorine for oxidizing manganese in a conventional filtration plant. The raw water quality at this site had TOC = 6–8 mg/L, pH = 6.5–7.2 and alkalinity 30–40 mg/L as CaCO3. The authors found that high ozone doses were required to oxidize Mn(II) in the raw water and that the oxidized particles formed small pinfloc that was difficult to settle and filter. Ozone was then applied to the settled water. Applying an ozone does of 2.5 mg/L to the settled water followed by filtration achieved 95% removal of dissolved Mn(II) (initial Mn(II) 0.6–1.0 mg/L). Other authors have reported that oxidation of Mn(II) creates colloidal MnOx(s) particulates which are difficult to remove following settling, and filter aids are needed to capture the solids at the filtration stage (O’Brien et al., 1996; Tobiason et al., 2008). In addition, chlorine addition prior to filtration is often not practiced if ozonation is being used at this step which eliminates the potential for adsorption/oxidation reactions within the filter. These factors make it difficult for ozone treatment to achieve residual dissolved Mn(II) levels below 0.025 mg/L (Tobiason et al., 2008).
7.2.2.4 Chlorine and oxygen
Direct chemical oxidation of Mn(II) by chlorine and oxygen is generally not used in drinking water treatment plants due to slow reaction kinetics and insufficient contact times unless alkaline pH conditions are present (pH > 8–9) (Kohl and Medlar, 2006; Brandhuber et al., 2013). Knocke et al. (1990a) reported that dosages 4 times greater than the stoichiometric requirements for free chlorine and a minimum contact time of 3 hours were needed to lower an initial Mn(II) concentration of 1.0 mg/L to 0.7 mg/L at pH 7.0 and a temperature of 25°C. When pH was increased to 9.0, Mn(II) was oxidized within one hour to below 0.05 mg/L. Lower temperatures (14°C) and the presence of DOC also increased reaction times significantly. An additional consideration with chlorine is that elevated dissolved Mn(II) concentrations leaving a treatment plant can be oxidized by the free chlorine residual in the distribution system due to longer contact times resulting in the creation of MnOx(s). This can lead to consumer complaints due to water discolouration (Kohl and Medlar, 2006; Brandhuber et al., 2013). As discussed previously, although chlorine is not effective for direct oxidation of dissolved Mn(II) under typical treatment plant operations, it is an integral component of the effective operation of certain adsorption/oxidation processes discussed is section 7.2.3 (Brandhuber et al., 2013).
DO is capable of oxidizing dissolved Mn(II); however, as with chlorine, the kinetics of the reaction are slow unless the pH of the water is above 9.0 to 9.5. These alkaline conditions are not present in most drinking water treatment processes, with the exception of lime or lime-soda ash softening treatment plants.
7.2.3 Adsorption/oxidation
Treatment of dissolved Mn(II) using adsorption and oxidation is based on the presence of MnOx(s)s MnOx(s) as coatings on filter media which are capable of adsorbing dissolved Mn(II) in the feed water and retaining it in the filter bed. The oxide surface then acts as a catalyst for oxidation of the adsorbed Mn(II) by oxidants (e.g., chlorine or permanganate) dosed into the water so that more oxides are produced . Several types of filter media are available that have the capability to adsorb dissolved Mn(II). These include traditional manganese greensand, pyrolusite and conventional filter media with MnOx(s) coatings that are commercially applied by the media supplier. Continuous or intermittent dosing of an oxidant to the raw water prior to contact with the media is required to maintain the MnOx(s) adsorption sites (Knocke et al., 1990b; Tobiason et al., 2008; Islam et al., 2010). The location of this process within a treatment plant can vary. For surface water treatment plants that chlorinate prior to filtration, it is often part of the existing particle removal filtration process. When pre-filter chlorination is not practiced, an adsorptive contactor unit can be placed following filtration (Knocke et al., 2010). For groundwater treatment plants, adsorption/filtration is often a process that is installed specifically for manganese and/or iron removal. A key benefit to having MnOx(s)-coated media as part of the treatment train is the ability of this process to achieve consistently low (< 0.015 mg/L) treated water manganese concentrations (Brandhuber et al., 2013).
7.2.3.1 Manganese greensand
Traditional manganese greensand is a granular filter medium processed from glauconite sand. Glauconite is synthetically coated with a thin layer of manganese base material (manganous ions) which is then converted to a MnOx(s) coating by conditioning the greensand in a potassium permanganate or chlorine solution (Knocke et al., 1990b; Sommerfield, 1999). This media has a large adsorptive capacity for removing dissolved Mn(II) (1.5 kg/m3). Greensand media is typically smaller (effective size 0.30 to 0.35 mm) than silica sand so it is good at capturing small particles. Since the head loss generated is higher than an equivalent bed depth of silica sand, most applications of greensand use pressure filtration (Brandhuber et al., 2013). In addition, greensand filters are best applied in groundwater systems with iron and manganese concentrations below 5 mg/L (Kohl and Medlar, 2006).
There are two standard approaches in which greensand filtration can be used to remove manganese: continuous and intermittent regeneration. Continuous regeneration involves feeding an oxidant (potassium permanganate or chlorine) or a combination of two oxidants into the raw water prior to contact with the greensand media. When potassium permanganate is used as the oxidant, most of the dissolved Mn(II) in the source water will be directly oxidized to MnOx(s) and the greensand media will function primarily as a physical removal process. If, however, any dissolved Mn(II) reaches the greensand media, it can be removed via adsorption onto the MnOx(s) surface coating. In these cases, the adsorptive capacity of the greensand is depleted more slowly since most of the manganese entering the filter is already oxidized. Backwashing to remove the MnOx particulates is conducted once head loss has reached a specified level (Knocke et al., 1990). An excess of oxidant is required to ensure the MnOx(s) adsorption sites are regenerated. Utilities should be aware that continuously dosing greensand with potassium permanganate can result in discolouration of the treated water due to excess permanganate present in the process (Brandhuber et al., 2013). Lower doses of potassium permanganate combined with chlorine or chlorine alone can also be used to continuously regenerate greensand media. Maintaining a free chlorine concentration of 0.5 to 1.0 mg/L across the media results in regeneration of MnOx(s) coatings on the media by oxidizing the adsorbed Mn(II). Brandhuber et al. (2013) indicated that the use of free chlorine is a suitable alternative for regeneration of greensand media due to lower chemical costs, less solids loading in the filter and the elimination of the risk of discolouration of treated water from the presence of excess permanganate. Free chlorine can regenerate the MnOx(s) surface on the media by oxidizing the adsorbed Mn(II). In cases where Mn(II) concentrations are greater than 1 mg/L in the source water or where the formation of disinfectant by-products (DBPs) needs to be decreased, then potassium permanganate may be the preferred or primary oxidant employed, with reduced levels of free chlorine being applied.
In an intermittent regeneration process, dissolved Mn(II) is removed by greensand media through adsorption onto the MnOx(s) coating on the greensand media. As the adsorption sites become filled, the media starts to lose its capacity to remove dissolved Mn(II). The media then needs to be taken offline so that an oxidant can be applied to oxidize the adsorbed Mn(II) and create new adsorption sites. This method requires careful monitoring of Mn(II) in the treated water to ensure that the greensand media is taken offline and regenerated prior to significant amount of dissolved Mn(II) passing through the filter and into the finished water.
Kohl and Medlar (2006) reported data from four groundwater treatment plants using manganese greensand filtration. These plants achieved manganese removals between 86% and 100% from average influent concentrations ranging from 0.35 mg/L to 0.52 mg/L to average treated water concentrations below 0.020 mg/L.
In addition to granular filter material manufactured from glauconite, several manufacturers also produce anthracite coal and silica sand media with a MnOx(s) coating applied to it through chemical processing. This media removes Mn(II) by the same mechanisms as greensand media. Recently, the supply of glauconite has been limited and these alternative media are viable options for water treatment plants using adsorption/oxidation treatment technology.
7.2.3.2 MnOx(s) coatings on conventional filter media
An important and often overlooked treatment method for Mn(II) removal is the adsorptive uptake of Mn(II) onto MnOx(s) coatings that have developed within a treatment plant on anthracite coal or silica sand filter media due to the presence of dissolved Mn(II) and free chlorine across the filter bed (Knocke et al., 1988, 1990b). In this process, the adsorbed Mn(II) is subsequently oxidized by the presence of free chlorine across the filter to create new MnOx(s) adsorption sites (e.g., continuously regenerated). In addition, only partial removal of the MnOx(s) coating occurs during backwashing, resulting in a net increase in MnOx(s) adsorption sites over time of operation (Hargette and Knocke, 2001). This process has been referred to as a “natural greensand effect” by Knocke et al. (1988) or “induced oxide-coated media effect (IOCME)” by Kohl and Medlar (2006). In many treatment plants, this MnOx(s) coated media process initiates and sustains itself without process engineers or operators being aware that it is occurring (Kohl and Melar, 2006; Brandhuber et al., 2013).
Several research studies have been conducted to better understand the factors that contribute to the effective removal of Mn(II) in treatment plants under MnOx(s) coated media filtration conditions. The effectiveness of MnOx(s) coated media filtration is dependent on the number and oxidation state of adsorption sites on the media, pH of the water, and the concentration of free chlorine across the filter (Knocke et al., 1988, 1990b, 1991; Hargette and Knocke, 2001; Tobiason et al., 2008). The key operational conditions for this process to function properly were summarized by Brandhuber et al. (2013). They include a free chlorine concentration in the filtered water of 0.5 to 1.0 mg/L (to ensure effective MnOx(s) coating regeneration) and a filter applied pH of 6.0 or higher, as lower pH levels result in competition for Mn(II) adsorption sites from H+ ions. In addition, manganese should be in the dissolved Mn(II) form entering the filter, since particulate Mn(IV) will not be adsorbed onto the media. Brandhuber et al. (2013) noted that under these conditions, drinking water treatment plants are routinely able to achieve very low treated water manganese concentrations (< 0.015 mg/L) even when pre-filter manganese concentrations are as high as 0.5 mg/L.
Tobiason et al. (2008) conducted research on MnOx(s) coated anthracite coal and silica sand filter media obtained from full-scale water treatment facilities. Results indicated that MnOx(s) coating levels were greatest for the media first exposed to free chlorine and Mn(II) as water passes through the filter (e.g., top of a dual media filter) regardless of the material type (anthracite and sand). Therefore, Mn(II) uptake occurs in the upper portion of a filter bed. The study also found that while typical free chlorine residuals used in a treatment plant are capable of partially regenerating MnOx(s) adsorption sites, the manganese removal capacity decreases over time. It was suggested that utilities could occasionally use offline intermittent regeneration of media, using concentrated chlorine or potassium permanganate solutions to improve the adsorptive capacity of media.
One of the main considerations with the use of MnOx(s) coated media filtration for manganese removal is the potential for the formation of DBPs. Tobiason et al. (2008) demonstrated that use of continuous pre-filter chlorination and MnOx(s) coated media for manganese control can cause increases of 10 to 50% in levels of distribution system DBPs (THMs and HAAs) in comparison to operation with post-filter chlorination only.
Over the last decade, changes to drinking water regulations for DBPs in many jurisdictions have led many utilities to move the application point of chlorine downstream of filters. This process change eliminates the presence of a free chlorine residual across the filters and therefore, also eliminates the ability to control manganese by MnOx(s) coated media filtration. Several authors have demonstrated that previously accumulated manganese on MnOx(s) coated filter media will release and cause elevated manganese concentrations in treated water during the transition to biological filtration (Gabelich et al., 2006; Tobiason et al., 2008; Kohl and Dixon, 2012). Utilities that are considering moving the application point of chlorine to a point following filtration should assess the potential for filter media to release manganese. Manganese release from filter media has been shown to occur in systems with different levels and sources of manganese (e.g., not only in systems with high manganese concentrations in the source water). Examples of these include systems with low but constant levels of manganese in the source water and systems using coagulants (e.g., ferric chloride) with manganese impurities. Kohl and Dixon (2012) conducted pilot testing of a 23 L/min conventional filtration system using filter media taken from a full-scale treatment plant with a well-established uniform MnOx(s) coating on the filter media. When the filters were operated without chlorine, manganese began leaching from the filters, resulting in increases of up to 0.03 mg/L in the total manganese concentration of the filtered water for up to 30 days. Similarly, Gabelich et al. (2006) reported manganese leaching from full-scale filter media within hours of removing chlorine from the filters (e.g., converting to biological filtration). The source of manganese was determined to originate from impurities in the ferric chloride coagulant used in the plant combined with the presence of free chlorine across the filters, resulting in an accumulation of MnOx(s) on the filter media. The utility reported an increase of 70–110 µg/L of total manganese leaving the plant following removal of chlorine across the filters. More detailed testing demonstrated that dissolution of manganese from filter media is a complex process in which three chemical forms of manganese interchange in the upper meter of filter media. Coagulant selection and solution pH were found to be important factors affecting the mobility of manganese in the filter media.
In cases where there is a need to limit the amount of pre-filter chlorine to control the formation of DBPs, post-filtration MnOx(s) media contactors can be a suitable manganese treatment (Tobiason et al., 2008; Knocke et al., 2010; Bierlein et al., 2015). Knocke et al. (2010) demonstrated that a MnOx(s) coated media adsorptive contactor effectively removed dissolved Mn(II) over a wide range of solution pH values (6.3 to 8.0), free chlorine levels ( > 1.0 mg/L), initial Mn(II) concentrations (0.03 to 0.3 mg/L) and hydraulic loading rates (up to 24 gpm/ft2).
As noted above, the effective removal of manganese using MnOx(s) coated media filtration in full-scale plants has been demonstrated in a number of studies (Charlton et al., 2002; Kohl et al., 2002; Herzner et al., 2003; Angara et al., 2004; Kohl and Medlar, 2006, Tobiason et al., 2007, 2008). In a survey of several plants relying on MnOx(s) coated media filtration, the average treated water concentration of all of the plants was 0.007 mg/L. The percentage removal in these plants ranged from 98% to 99% with average treated water concentrations for each plant ranging from 0.001 mg/L to 0.014 mg/L (Kohl and Medlar, 2006). Jeffcoat et al. (2007) reported that moving the location of the pre-chlorine addition from the rapid mix basin to the filter influent (creating MnOx(s) coated filter media) in a full-scale conventional treatment plant was an effective method for removing manganese. Influent manganese concentrations of approximately 0.05 mg/L were lowered to less than 0.01 mg/L using this process.
Tobiason et al. (2008) and Brandhuber et al. (2013) reported data from a number of case studies of utilities using MnOx(s) coated media filtration for control of dissolved Mn(II). Tobiason et al. (2008) demonstrated that a 25 MGD plant using alum coagulation, flocculation, settling, dual media filtration and disinfection with free chlorine was able to decrease pre-filter chlorine levels without compromising Mn(II) removal. The pre-filter chlorine dose was decreased from 2.8 to 1.6 mg/L, resulting in a decrease in the post-filtration chlorine residual from 0.9 to 0.2 mg/L. Treated water Mn(II) concentrations remained below 0.020 mg/L throughout this change despite raw water concentrations from 0.1 to 0.7 mg/L.
7.2.3.3 Pyrolusite
Manganese can be removed from solution by adsorption on a packed bed of granular pyrolusite, the mineral form of MnO2(s) (e.g., no coating required). Pyrolusite filters for drinking water treatment are typically a blend of sand and pyrolusite, with the ratio of sand to pyrolusite varying depending on the concentration of manganese in the source water. The addition of chlorine to the source water prior to the filter for continuous regeneration of the pyrolusite media is typical. However, intermittent regeneration using chlorinated backwash water can also be effective. An advantage to using pyrolusite filter media is that higher filtration rates are possible than with greensand processes, resulting in filter sizes and construction costs that are lower (Sommerfield, 1999, Casale et al., 2002). However, pyrolusite media have a very high specific gravity (> 4.0), and so require substantially higher hydraulic loading rates during backwashing operations in order to fluidize the media bed.
Limited information is available on the effectiveness of pyrolusite for manganese removal. Data reported by Kohl and Medlar (2006) indicate that pyrolusite was effective at removing between 81 and 99% of manganese at three groundwater treatment plants. These plants achieved average treated water manganese concentrations between 0.001 mg/L and 0.024 mg/L.
An alternative use of pyrolusite for dissolved Mn(II) control was reported by Kenari and Barbeau (2014), who utilized a fluidized-bed reactor as the means of contacting the water with the pyrolusite media. Test results showed nearly 100% removal of dissolved Mn(II) (initial manganese concentrations up to 0.3 mg/L) using contact times with the media that were one minute or less. Free chlorine was used as the oxidant of choice for regenerating the pyrolusite media surface within the fluidized bed. Hydraulic loading rates to the bed between 24 to 63 m/hr produced highly effective dissolved Mn(II) removal (pH between 6.2 and 7.8).
7.2.4 Biological filtration
Removal of manganese using biological filtration relies on the ability of naturally occurring manganese-oxidizing bacteria (MOB) present in biofilms on filter media to adsorb and oxidize dissolved Mn(II) and form particulate Mn(IV), which can then be removed by backwashing. Many different strains of bacteria have been identified in the literature as having the capability of oxidizing Mn(II); examples include Leptothrix, Sphaerotilus, Crenothrix, and Metallogenium (Mouchet, 1992). Research has indicated that the mechanisms of Mn(II) removal by MOB include intercellular oxidation by enzymatic action, adsorption at the surface of the cell membrane, and extracellular oxidation by catalytic action of excreted polymers. Adsorption to MnOx(s) solids that accumulate in the filter during the oxidation process also contributes significantly to Mn(II) removal in biofiltration (Casale et al., 2002; Burger et al., 2008a, Sahabi et al., 2009; Kohl and Dixon, 2012; Brandhuber et al., 2013; Bruins et al., 2015).
There are several biological and physicochemical factors that influence the performance of biological filters for manganese removal. Biological factors include the presence and diversity of MOB in the source water, the ability of MOB to form an active biofilm under the operating conditions of the filter, acclimation period of the MOB population, and maintaining biological activity after backwashing or other stresses to the filter. Acclimation periods for MOB range from 14 to 100 days with shorter periods observed following seeding of filters and when the temperature is between 20 and 30oC (Mouchet et al., 1992; Holst, 2004).
The important physicochemical factors are pH, redox potential, initial Mn(II) concentration and filter loading rates (Kohl and Dixon, 2012). The operating conditions required to establish an adequate MOB population in filters include maintaining aerobic conditions with a minimum dissolved oxygen (DO) level of 5 mg/L, a redox potential of 300 to 400 mV, and pH equal to or greater than 6.3 (Mouchet al., 1992; Burger et al., 2008a; Hoyland et al., 2014). The presence of ammonia, nitrite, and sulphide in the source water negatively impacts the ability of MOB to oxidize manganese. It has also been noted that groundwater is typically better suited to biofiltration for manganese removal since it has relatively stable influent manganese levels, temperature and pH. In contrast, surface waters can have large fluctuations in manganese levels and temperature and it is uncertain if MOB remains viable in a biofilter under these conditions for extended periods of time (Brandhuber et al., 2013).
Many full-scale biofiltration treatment plants that have been successful at removing manganese from groundwater have been reported in the literature (Mouchet et al., 1992; Li et al., 2005; Burger et al., 2008b; Kohl and Dixon, 2012). Kohl and Dixon (2012) reported data from 8 treatment plants in Canada, Europe and China using downflow mono-medium sand biofilters. These treatment plants were capable of greater than 93% removal of manganese to achieve treated water concentrations below the method detection limit of 0.03 mg/L. Several of the plants discussed above are located in New Brunswick and were reported in Burger et al. (2008b). The treatment train for these plants consists of water pumped from an aquifer into a downflow filter with 1,325 L/min design capacity and a filter unit with an outer diameter of 2.1 m, a filter bed depth of 1.8 m and a surface area of 3.4 m2. Compressed air is pumped into the filters to ensure a high ORP is achieved. The filters are backwashed at a rate of 20 m/hr at a frequency of approximately every 300 hours of operation. After backwashing, the filters are ripened for 5 minutes before being returned to service. Influent concentrations of manganese ranged from 0.86 to 1.39 mg/L and > 99% removal of manganese was achieved (< 0.03 mg/L in the effluent) in these plants. The pH of the source waters ranged from 6.46 to 7.63 and temperature was 9 to 10°C.
To date the majority of manganese biofiltration plants have been installed for treatment of groundwater sources. Recently, research has focussed on the feasibility of biofiltration for manganese removal from surface water supplies (Kohl and Dixon, 2012; Granger et al., 2014; Hoyland et al., 2014). Bench-scale studies were conducted by Hoyland et al. (2014) to evaluate the potential for removal of both NOM and Mn(II) by biofiltration under conditions representative of surface water treatment plants using coagulation and ozonation prior to filtration. The study demonstrated that MOB can achieve greater than 98% removal of Mn(II) in a biofilter with pH as low as 6.3. Stress tests further supported that well acclimated MOB (50 days) were successfully able to remove Mn(II) over a range of influent concentrations (0.1–0.2 mg/L), hydraulic loading rates (1.3 ×10-3 – 2.72 x 10-3 m/s), and temperatures (7–22°C) for a period of 1–2 days. Kohl and Dixon (2012) reported data for manganese removal in a 454 ML/d surface water treatment plant employing intermediate ozonation and 14 dual-media filters with 180 cm of GAC over 25 cm of sand. Ozone was applied after sedimentation before filtration at a dose of 1.5 to 2.0 mg/L and the pH entering the filters was 7.2 to 7.6. The filter influent total manganese concentrations ranged from 0.032 to 0.089 mg/L. The treated water manganese concentration was less than 0.002 mg/L (MDL) in all samples, demonstrating that manganese removals greater than 94% were achieved. In contrast, much lower removals were reported for a 190 ML/d intermediate ozonation treatment plant using 6-feet-deep granular activated carbon (GAC) filters. The filter influent total manganese concentration ranged from 0.018 to 0.071 mg/L. On average the filters removed 40% of the total manganese but large variations (21 to 76%) were observed on different sampling dates. The authors did not investigate the cause of the difference in performance between the two treatment plants.
As discussed in section 7.2.3.2, an important consideration for utilities that are considering transitioning from MnOx(s) coated media filtration to biological filtration is the potential for release of previously accumulated manganese on the filter media once the free chlorine residual across the filters is terminated (Gabelich et al., 2006; Kohl and Dixon, 2012). It is recommended that utilities intending to convert an existing MnOx(s) coated media filter consider removing the media and cleaning or replacing it prior to converting to biological filtration (Kohl and Dixon, 2012).
7.2.5 Softening and ion exchange
Treatment plants that use lime or soda ash softening can also achieve manganese removal by raising the pH of the water (e.g., >9.5–10) above the solubility of various manganese hydroxide and carbonate solid phases. Likewise, the elevated pH present in lime or lime/soda ash softening will greatly increase the rate at which dissolved Mn(II) is oxidized in the presence of DO. Where DO is present, formation of oxidized MnOx(s) solids will occur. Raising the pH of the source water to achieve dissolved Mn(II) removal is not a cost-effective treatment approach by itself; rather, this treatment method is typically used only if softening of the source water is also required. Many lime or soda ash softening facilities achieve highly effective manganese removal (and iron removal) as a by-product of the chemical softening reactions. Furthermore, the plant personnel often do not proactively operate the treatment system for achieving manganese removal. In some cases, manganese removal occurs in softening treatment plants without the operator’s knowledge, particularly when manganese is not being routinely monitored in source and treated water. A full-scale lime softening treatment plant reported lowering the average manganese concentration in the source water from 0.520 mg/L down to an average treated water concentration of 0.001 mg/L (Kohl and Medlar, 2006).
Dissolved Mn(II) can also be removed through cation exchange in zeolite softening processes. As with other cation exchange processes, backwashing the zeolite, typically with a brine solution, removes the manganese (as well as iron, calcium and magnesium) accumulated on the resin. One concern associated with zeolite softening as a means of dissolved Mn(II) removal is the presence of oxygen or another oxidant. Such conditions can result in oxidation of the exchanged Mn(II) to MnOx(s) on the zeolite surface, which in turn can decrease the overall cation exchange capacity of the zeolite resin over time (Sommerfield, 1999).
7.2.6 Sequestration
Sequestration is a control measure to limit the aesthetic water quality problems associated with oxidation of dissolved Mn(II) to form MnOx(s) particles within the distribution system. In general, sequestration is considered a temporary control measure for manganese because the sequestrant’s effect is time limited (Kohl and Medlar, 2006). Since sequestration does not remove manganese from the water, it does not limit exposure to manganese from drinking water. As such, only for water supplies with manganese levels below the MAC in the source or treated water should it be considered as a control option for reducing the potential for discoloured water; note, however, that there is a need to maintain a continuous dose over the long term. In most cases, the most appropriate method for reducing dissolved Mn(II) concentrations at the tap is installation or optimization of manganese treatment systems to maintain concentrations well below the MAC and AO so as to limit the amount of manganese accumulation in the distribution system. Very small groundwater systems with low levels of dissolved Mn(II) in the source water and limited resources may find the use of a sequestrant to control coloured water events to be the most practical option (AWWA, 1993). However, careful consideration needs to be given to the potential for the accumulation and subsequent release of manganese in the distribution system.
During chemical sequestration, a chemical agent is used to bind to dissolved manganese in order to create a solution phase complex in which manganese is not available for oxidation or precipitation. The addition of polyphosphates alone or in conjunction with chlorine is the most commonly reported methods used to sequester manganese (Sommerfield, 1999; Kohl and Medlar, 2006). The proper dosage of sequestering chemicals must be determined based on water quality characteristics and the manufacturer’s recommendations. Establishing the correct chemistry is often difficult due to changing water quality (i.e., variability of concentrations of manganese and other cations) and conditions in the distribution system (i.e., varying detention times and temperatures) (Kohl and Medlar, 2006). In addition, hydrolization of polyphosphates occurs over time and can result in the release of previously sequestered dissolved Mn(II). The rate of hydrolization increases at either low or high pH and increases with water temperature (Brandhuber et al., 2013). When sequestration is used as a control measure, routine sampling in the distribution system to measure total manganese concentrations is needed to ensure that the concentration remains below the MAC.
7.2.7 Other considerations
In addition to manganese present in source water, chemical addition and treatment plant processes can contribute to the amount of manganese that a treatment plant needs to manage. The three main sources of manganese from treatment plant operations are:
- presence of manganese impurities in coagulants (principally ferric-based coagulants) used for particle destabilization;
- resolubilization of Mn(II) from reduction of MnOx(s) solids stored in sedimentation basins as a result of anoxic conditions in the basin;
- presence of dissolved manganese in recycle streams from solids processing operations (Tobiason et al., 2008).
Kohl and Medlar (2006) summarized the literature that indicates the potential for contamination of treated drinking water by ferric salts that may contain manganese. Some studies demonstrate that in cases where a treatment plant uses enhanced coagulation, manganese contamination of the ferric chloride can result in soluble manganese concentrations up to 0.5 mg/L. Typical levels of manganese attributable to coagulant addition in surface water treatment plants range from 0.025 to 0.055 mg/L. Release from solids in sedimentation basins typically ranges from 0.01 to 0.10 mg/L with variations observed seasonally and manganese in residuals recycle streams can range from 0.01 to 1 mg/L (Tobiason et al., 2008). Thus, while the total flow from residual processing side streams can be relatively small in comparison to the influent flow to the treatment plant the total mass loading of dissolved Mn(II) from such side streams can be quite significant. Careful sampling of dissolved Mn(II) concentrations in these residuals processing side streams is strongly recommended. Likewise, treatment of such side streams for dissolved Mn(II) removal should be considered. Brandhuber et al. (2013) reported a case study where one utility constructed a full-scale, MnOx(s)-coated media filter for treating their liquid side stream from the residuals processing facility prior to discharge of that side stream into the plant’s terminal reservoir (which served as the source water to the facility). The dissolved Mn(II) concentration in the untreated side stream waters ranged from 0.02 mg/L to values in excess of 1 mg/L. Passage of the side stream waters through the bed of MnOx(s)-coated media yielded an average removal of greater than 90% dissolved Mn(II). Over time, the utility was able to demonstrate that treatment of this residuals processing side stream prior to release to their terminal reservoir resulted in a substantial decrease in the dissolved Mn(II) concentrations entering the treatment facility. This resulted in fewer operational challenges in addressing manganese issues, while also generating cost savings due to the reduced need for oxidant chemicals (e.g., permanganate) for treating the source water.
7.3 Distribution system
Manganese concentrations above 0.02 mg/L can cause complaints about discoloured water, plumbing fixture and laundry staining, and general dissatisfaction with the water quality (Sommerfield, 1999; Casale et al., 2002; Kohl and Medlar, 2006; Tobiason et al., 2008). In some cases manganese problems at the tap are not associated with high manganese concentrations in the source water. Rather, they are the result of the release of accumulated manganese in the distribution system from low but constant manganese present in the source or treated water. These manganese deposits can periodically release as a result of physical or hydraulic disturbances or unstable water chemistry and enter the distribution system water, resulting in discoloured water complaints.
In addition to the aesthetic concerns, manganese can act as an effective scavenger and sink for undesirable metals and inhibit the formation of stable Pb(IV) scale in lead pipe (Schock et al., 2014), creating other potential health risks due to possible release of co-occurring inorganic contaminants (Schock and Holm, 2003; Shock, 2005). An extensive review of the literature conducted by Kohl and Medlar (2006) indicated that manganese can be deposited in distribution systems even when the concentration leaving the treatment plant is as low as 0.02 mg/L. Therefore, a treated water goal of ≤ 0.015 mg/L of manganese in treated water is recommended (Brandhuber et al., 2013).
Utilities should ensure that manganese treatment and control measures include consideration of the deposition and possible release of manganese in the distribution system. In many cases, minimizing the manganese concentrations to as low possible before distribution is the most effective strategy.
7.3.1 Manganese accumulation and release in distribution systems
Manganese deposition can occur as a physical process, as a result of particle settling (U.S. EPA, 2006), through physical-chemical sorption (Friedman et al., 2010) and through biological accumulation (Sly et al., 1990; Cerrato et al., 2010; Ginige et al., 2011). Manganese has been shown to accumulate in loose deposits of distribution pipe materials including iron (Zacheus et al., 2001; Cerrato et al., 2006; Peng and Korshin, 2011), polyethylene (Zacheus et al., 2001), polyvinyl chloride (Cerrato et al., 2006) and lead (Schock et al., 2014) pipes. Cerrato et al. (2006) found that manganese accumulated to a lesser extent in iron pipes (3.48% by weight) compared to PVC pipes (6% by weight). Cerrato et al. (2006) found that in the iron pipes, manganese was associated with tubercle deposits; whereas manganese accumulated in PVC pipes, resulted in easily removed/released surface deposits. Manganese concentration in distributed water decreases as distance from the water treatment plant increases (Kohl and Medlar, 2006). Barbeau et al. (2011) observed that manganese decreased as water age increased, with a 37% decrease in manganese concentration after a residence time of 24 hours. Notably, in Brandhuber et al. (2015) estimates were made for manganese stored on distribution system pipes based on data collected in Friedman et al. (2010). Deposited manganese ranged from 0.1 mg/ft2 to 10,000 mg/ft2, with an estimated median of 210 mg/ft2, equivalent to approximately 3.8 lbs Mn/mile (based on 6-inch-diameter pipe) or 7.7 lbs Mn/mile (based on a 12-inch-diameter pipe). Brandhuber et al. (2015) also noted that only 1.5% of the manganese deposit would need to be released to exceed the U.S. EPA Health Advisory values of 1 mg Mn/L for both 1 day and 10 days. Peng and Korshin (2011) found that manganese was significantly associated with the organic and mobile fractions of the corrosion scale, and the majority of the manganese was found to be in the dissolved fraction. The authors also found that 9% of manganese was in the exchangeable fraction which is prone to displacement by competing ions, thus making manganese deposits susceptible to changes in water quality.
Physical disturbances to the distribution system can also cause the release of scale deposits containing manganese. For example, Del Toral et al. (2003) documented an association between disturbances events, such as meter installation/replacement, autometer reader installation, leak repair, external service shut-off valve repair/replacement and significant street excavation, and increased lead release.
Manganese can also accumulate in distribution system biofilms (Ginige et al., 2011; Cerrato et al., 2010; Sly et al., 1990). Both manganese oxidizing (Cerrato et al., 2010; Sly et al., 1990) and manganese reducing (Cerrato et al., 2010) bacteria have been identified. The entrapped manganese accumulated in drinking water biofilms has been shown to be susceptible to release during biofilm inactivation (Ginige et al., 2011). In bench-scale experiments by Ginige et al. (2011), biofilm reactors fed with either low (0.02 mg/L) or high (0.04 mg/L) influent levels of manganese were subjected to a 14-day biofilm inactivation treatment with 3 mg/L chlorine. In both the high and low level reactors, the biofilm ATP concentration decreased approximately ten-fold within 2 days. During inactivation, turbidity and manganese increased in both reactors. In the low influent reactor, manganese release peaked on day 4 at 0.07 mg/L and returned to baseline levels of 0.01 mg/L by day 8. In the high reactor, manganese release peaked on day 4 at 0.08 mg/L from 0.06 mg/L, and effluent manganese levels gradually decreased to 0.03 mg/L by day 12. The authors suggested that exopolymeric substances and the metal oxide/hydroxide layer could play a role in the detachment timing for manganese from biofilms.
7.3.2 Implications of manganese accumulation for other distribution system concerns
The presence of manganese in distribution systems also has implications for other metals of concern. Manganese accumulation on lead pipes can obstruct the formation of the more stable Pb (IV) corrosion scale, increasing risk of lead release through more readily soluble scales (Schock et al., 2014). MnOx(s) have been shown to be effective scavengers for a variety of heavy metals, including lead (Dong et al., 2003) and arsenic (Ouvrard et al., 2002). In Freidman et al. (2010), barium, lead, nickel, vanadium, arsenic, radium, chromium and uranium were shown to be associated with MnOx(s) deposits in distribution system pipe materials. Manganese deposits represent potential sinks for the accumulation and subsequent release of these metals. Schock et al. (2014) found that in a distribution system where manganese was controlled through a unidirectional flushing program, lead levels were also reduced as lead accumulation in the scale decreased in the absence of manganese.
Additionally, a link between the presence of particles in the distribution system and increased organic matter was observed (Vreeburg, 2010). In a study where additional treatment removed all particles (> 0.1 µm) entering the distribution system, levels of organic matter was found to be six times less than in the conventional distribution system where particles were present. Vreeburg (2010) suggested that the particles could offer increased surface area for biological regrowth.
7.3.3 Manganese management in distribution systems
In a review article on discolouration in distribution systems by Vreeburg and Boxall (2007), two pathways for managing discolouration risk in distribution systems were noted:
- adoption of a cleaning threshold to prevent the accumulation of material; and
- limiting or preventing particles from entering or being generated in the distribution system.
Similarly, Brandhuber et al. (2015) recommended a manganese management approach that minimized the amount of manganese entering the distribution system; minimized the manganese stored in the distribution system through best practices for main cleaning; minimized physical/hydraulic disturbances; and maintained stable water chemistry.
High velocity networks can be used as a self-cleaning method to minimize the accumulation of sediments (Vreeburg, 2010). Particles that accumulate during periods of low water demand can be resuspended by using sufficiently high water velocity, thus preventing long-term accumulation issues. The practicality of high velocity networks may be limited as they require compatible distribution system designs (branched vs. looped).
The practice of pigging pipes can also be used to remove sediments but caution must be used when employing pigging as it is not appropriate for all pipe and water quality types (FCM, 2003). For example, the pigging of unlined cast iron pipes was associated with greater iron and downstream lead particle release when compared to cast iron pipes that were lined and pigged and those that were untouched (i.e., unlined and not pigged ) (Camara et al., 2013).
Unidirectional flushing is considered a best practice for managing particles in a distribution system (FCM, 2003; Brandhuber et al., 2015). In practice, Zacheus et al. (2001) found that before flushing, manganese comprised 3.7% (by weight) of old soft deposits in distribution system pipes, whereas one year after flushing, manganese comprised only 0.58% (by weight). Similarly, Barbeau et al. (2005) found that loose deposits from both cement-lined ductile iron and unlined grey cast iron distribution system pipes were composed of 5.9% (by weight) manganese. One year following flushing, the manganese content had decreased to 0.35%. In a field study by Carrière et al. (2005), unidirectional flushing was found to be an effective method for the removal of loose distribution system deposits in some cases. The authors also cautioned that unidirectional flushing should be applied on site-specific basis. Vreeburg (2010) also recommended the “clear water front” approach, which requires that the upstream water which is used to flush pipes come from pipes with no re-suspendable material, so to prevent the introduction of sediment into the flushed pipe. In extreme cases, pipe replacement could be used as a last resort option for managing accumulated manganese in distribution systems (Brandhuber et al., 2015).
Maintaining stable water quality conditions is an important factor in minimizing release of accumulated manganese in the distribution system. Friedman et al. (2010) identified several key water quality conditions that should be controlled in order to maintain water stability for deposited inorganics, including pH, ORP, and corrosion control measures, as well as avoiding the uncontrolled blending of surface water and ground water, and avoiding the uncontrolled blending of chlorinated and chloraminated water.
Managing manganese accumulation and release may be a significant challenge for utilities, as even utilities meeting the aesthetic objective could have historical reserves of legacy manganese stored on distribution system pipes. Identifying high risk areas in the distribution system will require an understanding of factors affecting risk of accumulation (e.g., presence of manganese in source/treated water, proximity to water treatment plant, pipe materials, biofilm) as well as risk of release (e.g., unstable water quality conditions, hydraulic/physical disturbances). Distribution system areas where there is high risk for both accumulation and release may be the ideal locations for increased event-based monitoring.
7.4 Residential scale treatment
In cases where manganese removal is desired at the household level – for example, when a household obtains its drinking water from a private well – a residential drinking water treatment device may be an option for reducing manganese concentrations in drinking water. Before a treatment device is installed, the water should be tested to determine general water chemistry and verify the presence and concentrations of manganese in the source water. Periodic testing by an accredited laboratory should be conducted on both the water entering the treatment device and the finished water, to verify that the treatment device is effective. Treatment devices lose their removal capacity through usage and time and need to be maintained and/or replaced. Consumers should verify the expected longevity of the components in their treatment device according to the manufacturer’s recommendations and service it when required.
Health Canada does not recommend specific brands of drinking water treatment devices, but it strongly recommends that consumers use devices that have been certified by an accredited certification body as meeting the appropriate NSF International (NSF) /American National Standards Institute (ANSI) drinking water treatment unit standards. These standards have been designed to safeguard drinking water by helping to ensure the material safety and performance of products that come into contact with drinking water. NSF/ANSI Standard 42: Drinking Water Treatment Units – Aesthetic Effects includes reduction requirements for manganese. For a device to be certified for manganese reduction under Standard 42, it must be capable of achieving the reduction of 1–2 mg/L of dissolved manganese (Mn(II)) down to a maximum treated water concentration of 0.05 mg/L (NSF/ANSI, 2014a).
Certification organizations provide assurance that a product conforms to applicable standards and must be accredited by the Standards Council of Canada (SCC). In Canada, the following organizations have been accredited by the SCC to certify drinking water devices and materials as meeting NSF/ANSI standards (SCC, 2014):
- CSA Group;
- NSF International;
- Water Quality Association;
- UL LLC;
- Bureau de Normalisation du Québec; and
- International Association of Plumbing and Mechanical Officials.
- An up-to-date list of accredited certification organizations can be obtained from the SCC.
Although there are currently no treatment units certified specifically for manganese reduction, there are several treatment technologies that can be effective for manganese removal at the residential scale. Traditionally, manganese treatment systems have been installed at the point-of-entry (POE) into the home to reduce the potential for discoloured water and staining of laundry (i.e., aesthetic concerns). These systems are typically designed and constructed for residential use by drinking water system providers or dealers. Treatment technologies that can be used at the point-of-entry to reduce the likelihood of discoloured water and staining within the home are ion exchange (i.e., water softener) and greensand filtration. Drinking water treatment devices are also available to reduce manganese concentrations at point-of-use (POU) in a home (e.g., kitchen tap) or as pour-through filters (e.g., pitcher type of filter). Treatment technologies for manganese removal that are appropriate for residential treatment are reverse osmosis, ion exchange (including water softeners and other cation exchange systems), and oxidizing filters (e.g., greensand filters).
Health Canada strongly recommends that any chemicals and components used in these treatment systems be certified to NSF/ANSI Standard 60 – Drinking Water Treatment Chemicals Health Effects (NSF/ANSI, 2014b), NSF/ANSI Standard 61 – Drinking Water System Components Health Effects (NSF/ANSI, 2014c) and NSF/ANSI Standard 372 – Drinking Water System Components Lead Content (NSF/ANSI, 2011). Reverse osmosis systems are covered in NSF/ANSI Standard 58: Reverse Osmosis Drinking Water Treatment Systems (NSF/ANSI, 2014d) and water softeners are covered under NSF/ANSI Standard 44: Cation exchange water softeners (NSF/ANSI, 2014e).
Selection of the most effective treatment system for a household will depend on a variety of factors, including the concentration and form (dissolved or particulate) of manganese and other parameters such as hardness, iron, alkalinity, sulphide, ammonia and dissolved organic carbon that are present in the source water.
Reverse osmosis (RO) drinking water treatment systems have been shown to be the most effective and reliable POU treatment technology for reducing manganese concentrations in drinking water in households with a water supply containing elevated manganese concentrations (Barbeau et al., 2011; Brodeur and Barbeau, 2015; Health Canada, 2015). Barbeau et al. (2011) found that eight reverse osmosis systems installed in homes supplied by well water achieved close to 100% removal of manganese even in the presence of high manganese concentrations (> 1 mg/L). In a more detailed report prepared using the same data, Brodeur and Barbeau (2015) reported that POU reverse osmosis systems were capable of achieving treated water concentrations below 1.0 µg/L when manganese in the well water ranged between 141 and 3.9 µg/L (86–100% removal). Controlled laboratory testing has also been conducted on POU reverse osmosis systems to assess their ability to lower manganese concentrations at the tap (Health Canada, 2015). Three different POU reverse osmosis systems certified under NSF/ANSI Standard 58 for removal of other metals (i.e., arsenic, barium, chromium, copper, lead) were tested as part of this study. Testing was conducted according to the requirements outlined for inorganic chemical reduction claims under NSF/ANSI Standard 58. Two rounds of testing were conducted with different influent manganese concentrations. In the first round, the median influent manganese concentration to each RO system was 100 µg/L (representative of average well water concentrations). Two of the systems demonstrated median removals greater than 95%, achieving treated water concentrations below 4 µg/L. The third system, which was tested with the storage tank removed, had a median removal of 88% to achieve treated water concentrations below 24 µg/L. In the second round of testing, influent concentrations to the RO systems ranged between 1.5 and 3.5 mg/L (representative of maximum concentrations of manganese in well water). Results were similar to those achieved in the first round of testing with median removals for two of the systems of 95–97% to achieve median treated water concentrations between 63 and 100 µg/L. The system without the storage tank achieved a lower median removal of 91% and a median treated water concentration of 140 µg/L. Overall, under most conditions POU RO systems are capable of removing manganese to below the MAC. Well owners with high manganese concentrations (>2 mg/L) may need to combine two treatment technologies such as POE ion exchange followed by POU RO in order to achieve treated water concentrations below 100 µg/L.
There are also POU devices available that use cation exchange media which are effective for manganese removal. Carrière et al. (2011) found that pour-through filters composed of cation exchange (and GAC) media were effective at reducing an influent (incoming) manganese concentration of 1 mg/L by greater than 60% at 100% of their estimated treated water capacity. These filters were also often capable of up to 100% removal during the early operation of the filters. Cation exchange devices designed for under-the-sink installation were also found to be effective for dissolved manganese removal (up to 100%) but in some cases their effectiveness decreased after only 50% of their estimated treated water capacity. It is also important to note that the treated water concentrations increased when these units were operated beyond their estimated capacities. Since most cation exchange media used in drinking water treatment devices have a lower affinity for manganese than calcium, magnesium and other metals such as iron, manganese ions will be displaced off the resin more easily. This results in a lower operating capacity for manganese removal as well as the potential for increased manganese in the treated water (Carrière et al., 2011). Therefore, careful operation and monitoring of these systems is necessary.
Conventional water softeners that treat water entering the home also use ion exchange technology and have the potential to removal low levels of manganese. If particulate manganese is present in the source water, it may damage the resin and reduce the effectiveness of these systems. As discussed above, manganese removal efficiencies can be variable using ion exchange due to the selectivity of the resin towards cations that cause hardness (calcium and magnesium). Therefore, manganese removal using water softeners will be less effective when source water with high hardness or iron is being treated. Brodeur and Barbeau (2015) and Barbeau et al. (2011) observed a large range in the removal efficiencies (-48% to 99%) of ion exchange systems operating in residences treating groundwater. Despite the large ranges in manganese removals observed in this study, the systems were found to be effective overall. Seventy-five percent of the systems achieved 99% removal of manganese (influent concentrations up to 211 µg/L) to achieve treated water concentrations below 19 µg/L.
POE oxidizing filters such as greensand filters can be used for POE residential scale manganese removal. These systems consist of a filter media treated with potassium permanganate to form a coating that adsorbs and then oxidizes dissolved manganese. These filters are often referred to as manganese greensand filters. Greensand filters require significant maintenance, including frequent regeneration with an oxidant and regular backwashing to remove oxidized manganese particles. The preferred oxidant is chlorine, although potassium permanganate can also be effective. Deficient operation and maintenance of greensand filters has been associated with an increase in manganese concentrations in the tap water in homes treating well water for manganese (Barbeau et al., 2011). Brodeur and Barbeau (2015) reported data for three green filters installed in residences for manganese removal. None of the systems were capable of removing manganese below 100 µg/L. In addition, it was observed that two of the systems were releasing manganese from the filters, resulting in an increase in the treated water concentration of between 17 and 199%. For these reasons, homeowners need to give careful consideration to the selection and operation of these types of treatment systems for manganese removal. In addition, it is important to routinely monitor the manganese concentration in the water treated by greensand filtration to ensure that the system is effectively removing manganese and that release of manganese from the filters is not occurring.
Depending on the concentration of manganese in the source water and the type of treatment selected, households with a private well may want to treat water at the POE to reduce the likelihood of water discolouration and staining of laundry, in addition to treating at the POU to ensure that levels are reliably below the MAC in the home’s drinking water.
8.0 Kinetics and metabolism
The following section summarizes the data presented in several recent reviews (Aschner et al., 2005; Dorman et al., 2006; Health Canada, 2010) as well as more recently published papers. The reader is referred to these documents for more detailed information.
8.1 Absorption
Manganese is an essential nutrient that is subject to homeostatic control through both regulation of its absorption across the gastrointestinal (GI) tract and its hepatobiliary excretion (the so-called “first-pass effect”) following ingestion. In contrast, inhaled manganese occurring as a result of occupational exposures can bypass this homeostatic control on intestinal uptake and first-pass distribution to the liver, and be transported via the olfactory system or respiratory tract to the brain (Aschner et al., 2005; Roth, 2006). Thus, absorption of manganese via inhalation differs significantly from absorption through drinking water ingestion.
Dietary ingestion represents the largest source of oral exposure to manganese in humans. In addition to homeostatic control on uptake, absorption is influenced by the bioavailability and/or bioaccessibility of manganese in the GI tract, its chemical form, the amount of manganese ingested, and iron status, as well as gender and age. A smaller fraction of the manganese content from food and water is typically absorbed through the GI tract in adults compared to infants (Davidsson et al., 1988; Johnson et al., 1991; Finley et al., 1994; IOM, 2000; Health Canada, 2010). Oral studies in animals generally yield similar absorption results (Pollack et al., 1965; Davis et al., 1993; Finley et al., 1997; Zheng et al., 2000). Absorption of ingested manganese is higher in the neonatal period, and manganese is thought to be more bioavailable (Dorothy Klimis-Zacas, 1993). Neonates have been reported to have a higher retention of manganese, with formula-fed infants retaining approximately 20% of the oral intake (Aschner and Aschner, 2005).
A review of 13 published studies that used a wide variety of exposure scenarios in humans (without consideration of fasted state) found no statistically significant difference in the bioavailability (defined in the review as the fraction that enters into the systemic circulation, i.e., excluding the part excreted in the bile after the first-pass effect in the liver) of manganese when ingested in water compared to in food (food: water ratio was 0.7) (Ruoff, 1995). For food, the GI bioavailability was similar whether manganese was intrinsically present in the foods or extrinsically added to the diet. However, upon re-evaluation of the data for fasted individuals, the bioavailability of manganese in water was found to be roughly two-fold higher than in food (food: water ratio was 0.5) (Ruoff, 1995). Overall, these data suggest that the GI bioavailability of manganese in humans may partially depend on the interactions between manganese and materials (e.g., fiber, protein and other inorganic substances) present in the gut rather than on the bioavailability in each ingested media. Net manganese absorption from the diet was shown to be influenced by the presence of other trace minerals, cellulose, pectin, phytate or ascorbic acid, and other dietary constituents (Aschner et al., 2005) as well as the bioaccessibility of manganese in solid matrices (i.e., the proportion of manganese in a solid matrix that is potentially biologically available for absorption). Bioaccessibility was estimated at 55–95% in a variety of animal-based food (Lei et al., 2013) and at 12%–41% in 20 urban soils (Sialelli et al., 2010). In adult male rats, bioavailability was also reported to be similar following 61 days of equivalent manganese exposures in diet and drinking water (Foster et al., 2015). However, it should be noted that adaptive changes to control manganese levels (including reduced gastrointestinal absorption and increased excretion) which occur in adults following elevated exposure to manganese are not established in infants, who lack these homeostatic controls (Aschner et al., 2005; Foster et al., 2015).
The chemical form (i.e., species) and valence state of manganese affects its absorption. Soluble manganese (e.g., manganese chloride) is more readily absorbed (Roels et al., 1997), as is the complex-associated trivalent oxidation state of manganese found in human milk. This trivalent form binds to lactoferrin and is regulated across the GI tract via lactoferrin receptors in the brush border membranes of epithelial cells throughout the length of the small intestine. Infant formula contains the divalent oxidation state which does not bind to lactoferrin and therefore intestinal uptake cannot be regulated by lactoferrin receptors (Erikson et al., 2007b). In healthy adults, elevated dietary manganese levels were reported to be accompanied by a decrease in percent absorption, and enhanced hepatic metabolism and biliary and pancreatic elimination of manganese (Finley et al., 2003; Aschner et al., 2005).
In both humans and animals, low iron status has been shown to increase manganese absorption, independent of body stores of manganese (Mena et al., 1969; Chandra and Shukla, 1976; Shukla et al., 1976; Finley and Davis, 1999; Arnich et al., 2004). Absorption of both iron and manganese across the gut uses the same transport system, resulting in competition between manganese and non-heme iron at the level of the divalent metal transporter 1 (DMT-1, also known as DCT-1 or nramp-2) (Davis et al., 1992a) with expression of DMT-1 (mRNA levels) being strongly increased in the duodenum in response to iron depletion (Gunshin et al., 2001).
Gastrointestinal absorption of manganese is also influenced by individual factors such as gender and age. In humans, GI absorption of manganese was found to be higher in females than in males (Finley et al., 1994); this is possibly related to the lower iron status in and increased iron need in women. Increased absorption and retention have been observed in neonates and infants in both humans (up to an undetermined age) and rodents (up to 17–18 days age) (Gruden, 1977; Keen et al., 1986; Dörner et al., 1989; Kostial et al., 1989 ; Zlotkin et al., 1995; Aschner et al., 2005). Infants can absorb up to 40% of ingested manganese (compared to 3% absorption estimated in adults, making them particularly susceptible to effects resulting from elevated manganese exposure (Neal and Guilarte, 2013). The enhanced biliary retention of manganese in neonates can be explained by the immaturity of the hepatobiliary system (reduced excretion) (Miller et al., 1975; Cotzias et al., 1976) and by increased GI absorption (Cahill et al., 1980). Increased absorption at very young ages may also be a compensatory mechanism due to the increased metabolic needs of infants in comparison with adults, since manganese is required for adequate bone mineralization, as well as for connective tissue synthesis (Santamaria, 2008). Interindividual variability in manganese retention appears to be large: in human adults administered a dose of radiolabeled manganese in an infant formula, the mean absorption was 5.9 ±4.8%, and the range was 0.8–16%, i.e., a twenty-fold difference; retention measured on day 10 (0.6–9.2%) also indicated substantial differences between individuals (Davidsson et al., 1989a).
The dermal absorption of manganese is not well understood but is expected to be extremely limited (Aschner et al., 2005). No experimental data was identified regarding dermal absorption of manganese.
8.2 Distribution
Information regarding the distribution of manganese has been summarized in detail in reviews (Aschner et al., 2005; 2007) and a Health Canada (2010) report.
8.2.1 Tissue distribution
Manganese is distributed throughout the body via systemic circulation. Within the blood, manganese is present in plasma, where Mn(II) is bound to albumin, red blood cells, where it is mainly bound to hemoglobin (Gibbons et al., 1976; Saric, 1986; Davidsson et al., 1989b), and white blood cells.
The distribution of manganese depends on its chemical form (valence and solubility). In a dietary study, levels of manganese in the liver and kidney were significantly higher in male mice exposed to manganese acetate or manganese carbonate than in mice exposed to manganese chloride or manganese dioxide (Komura and Sakamoto, 1991).
Manganese concentrations in most human tissues range between 0.1 and 1 µg/g wet weight, but higher values have been reported in the liver, olfactory bulb, kidney and some regions of the brain in presumed normal adult subjects (Aschner et al., 2005). The highest concentrations of manganese tend to be reported in tissues with high mitochondria content, such as liver, pancreas, kidneys, pituitary gland and intestines; the lowest concentrations are reported in muscles, bones and fat (Merian et al., 2004; Health Canada, 2010). Bones represent the largest tissue store of manganese and account for 25–40% of total body burden because of bone mass not concentration (Aschner et al., 2005; Roth, 2006). Pregnancy was found to be associated with elevated levels of manganese in the liver of rats (Jarvinen and Ahlström, 1975).
In human milk, manganese concentrations are generally less than 30 µg/L, although values attaining 60 µg/L have been reported (Health Canada, 2010). Measured concentrations of manganese in milk were found to decrease from birth over seven days postpartum (Arnaud and Favier, 1995). Ratios of manganese concentrations in whole blood vs. breast milk were estimated in women with occupational and/or environmental exposure to metals in a region of India highly affected by steel industry, and in (control) women living 100 km away (Sharma and Pervez, 2005). The average ratios varied from 2.8 to 4.1 in exposed women, and from 1.7 to 2.5 in control women. Within both exposed groups, manganese concentrations in blood and milk were correlated. A study that investigated maternal exposure to elevated levels of manganese and other metals in drinking water in Bangladesh reported elevated manganese concentrations in urine, though no correlation between elevated manganese levels in drinking water and breast milk or blood was found (Ljung et al., 2009).
Fetuses, neonates and pups are exposed to (maternal) manganese since it crosses the placental barrier and is found in milk. Oral exposure of rat dams to manganese chloride in drinking water (2000 ppm) during gestation (272 mg Mn/kg bw per day) as well as during lactation (480 mg Mn/kg bw per day) was shown to increase six- to eight-fold the manganese total body burden (measured by atomic absorption spectometry) in neonates and 11-day-old pups. This increase was independent of age and exposure duration; a similar increase was reported when exposure occurred during gestation only, suckling only or both gestation and suckling (Kostial et al., 2005). Increased manganese concentrations were also reported in the forebrain and hindbrain of newborn rats after in utero exposure (Kontur and Fechter, 1985).
8.2.2 Distribution within the brain
The brain readily takes up manganese from the circulation, and levels may increase in the brain as a result of elevated exposures. The half-life of manganese in the brain was estimated to be 51–74 days in the rat, 53 days in the macaque monkey, and 53 days in humans (Cotzias et al., 1968; Newland et al., 1987; Takeda et al., 1995).
Recent reviews regarding the uptake of manganese into the brain led to the conclusion that manganese can enter the brain via several pathways, including the blood through capillary endothelial cells of the blood-brain barrier (BBB), from the blood through the cerebral spinal fluid (CSF) via the choroid plexuses, and, when exposure occurs by inhalation, from the nasal mucosa to the brain olfactory bulb via olfactory neural connections, though the latter is believed to be important only in cases of elevated nasal respiratory exposure (Aschner et al., 2005; Crossgrove and Yokel, 2005; Bock et al., 2008). However, it is not clear whether a particular one of these pathways predominates in cases of severe manganism or cases of subtle neurological impairment in humans, or what the predominant molecular mechanism(s) of transport are in any of these pathways (Aschner et al., 2005).
In animals, increased concentrations of manganese in the brain following acute or chronic oral exposure have been reported in numerous studies (Holbrook et al., 1975; Rehnberg et al., 1982; Kristensson et al., 1986; Newland et al., 1989; Chan et al., 1992; Davis et al., 1992b; Malecki et al., 1996; Lai et al., 1999). For instance, in rats exposed to manganese through drinking water (10 mg MnCl2•4H2O/mL) for five days, manganese concentrations were increased in some regions of the brain, with the highest concentration in the striatum and the lowest concentration in the cerebral cortex. After ten days, the highest concentrations were in the pons and medulla and the lowest concentration was in the hypothalamus. Variation in manganese content across brain regions was more pronounced in neonates than in weanling and adult rats (Chan et al., 1992). In the mature (PND 120) female offspring of Wistar rats exposed through gestation, through lactation and, from weaning onwards by direct exposure to manganese in drinking water (280, 2800 or 5600 mg Mn/L), dose-dependent increases in manganese concentration were observed in all brain regions (midbrain, pons and medulla, cerebellum, cerebral cortex and, more prominently, in hypothalamus, striatum and hippocampus), except in the cerebral cortex and hippocampus of rats treated with the lowest dose (Lai et al., 1999).
A difference in manganese brain distribution between neonatal and adult rats exposed to the same oral doses of manganese chloride (25 or 50 mg/kg bw per day) was reported in a study by Dorman et al. (2000). An increase in manganese concentration was observed only in the striatum and cerebellum in adult rats of the highest dose group, whereas neonatal exposure resulted in increased striatal, hippocampal, hindbrain, and cortical concentrations of manganese in all groups exposed. These results suggest that neonates may be at greater risk than adults for neurotoxicity resulting from high oral exposures to manganese.
Manganese-induced neurotoxicity is associated with elevated brain levels of manganese that occur following elevated exposure, with the striatum (caudate nucleus and putamen), globus pallidus, subthalamic nuclei (collectively the basal ganglia) and frontal cortical areas appearing particularly susceptible (Dobson et al., 2004). The human caudate/putamen and globus pallidus have been identified as primary neurotoxicity targets following exposure to manganese (Pal et al., 1999; Aschner et al., 2005).
8.2.3 Cellular uptake and efflux
Both Mn(II) and Mn(III) can cross the BBB (Aschner et al., 1999), with Mn(II) utilizing a number of different divalent cation transporters, while transferrin-associated Mn(III) is taken up via the transferrin receptor (Health Canada, 2010). Uptake of non-protein-bound Mn(II) appeared to be much more rapid than uptake of Mn(III)-transferrin (Tf ) complex; Mn(II) would thus be the predominant species entering the brain from plasma, while the Mn(III)-Tf complex uptake may play a lesser role in brain manganese uptake (Yokel et al., 2006). Little is known about transport mechanisms of manganese into the brain during the developmental period. DMT-1 and TfR are present in the developing brain, as shown by the expression of DMT-1 and TfR proteins in Sprague-Dawley rat pups as early as PND5 with concentrations that increase through PND15 in all regions examined (cortex, hippocampus, striatum) (Siddappa et al., 2002; Garcia et al., 2006).
Recently, the SLC30A10 cell-surface transporter has been shown to play an important role in manganese efflux from the brain (Leyva-Illades et al., 2014). The transporter has been shown to reduce cellular levels of Mn, and mutations of this transporter lead to manganese toxicity. A number of other transporters also help to maintain manganese homeostasis within the cell (Chen et al., 2015).
8.3 Metabolism
In its elemental form manganese does not undergo metabolism and is absorbed and excreted unchanged. Manganese is usually ingested in the form of ionized salts and can change valence states during or after absorption. Although the divalent form of manganese is predominant in biological systems, manganese can be present in the trivalent form in most enzymes; bound to transferrin (Tf); in human milk, bound to lactoferrin (Aschner et al., 2005).
In the liver and circulation, a proportion of divalent manganese is oxidized to the trivalent form and stabilized to protein ligands such as Tf (Aschner et al., 2005). Tf binds Mn(III) (or Fe(III)), and not Mn(II) (Aisen et al., 1969; Critchfield and Keen, 1992; Ueda et al., 1993). Oxidation of Mn(II) to Mn(III) may also occur intracellularly via reaction with free radical species such as the superoxide anion (Archibald and Tyree, 1987). While ceruloplasmin in circulating plasma has been suggested to catalyze the oxidation of Mn(II) to Mn(III), leading to a shift in manganese binding in vitro from α2-macroglobulin to Tf (Gibbons et al., 1976), recent studies in ceruloplasmin knock-out mice have shown clearly that ceruloplasmin is not necessary for plasma manganese oxidation and loading onto plasma Tf (Jurasa and Smith, 2009). In addition, it is possible that small amounts of Mn(II) may be spontaneously oxidized to form small amounts of Mn (III) which, even in trace amounts, can lead to the formation of reactive free radicals (HaMai et al., 2001). While studies have suggested that the majority of manganese in mammalian cells exists as Mn(II) (Reaney et al., 2002), accurate detection of manganese valence state in vivo is limited by a number of technological challenges.
Data suggest that the valence state of manganese is an important determinant for manganese retention and toxicity (Yokel et al., 2006). Mn(II)-α2-macroglobulin was cleared more rapidly from cows than Mn(III)-Tf (Gibbons et al., 1976). In vitro studies revealed a higher potential of cytotoxicity and neurotoxicity for Mn(III) than for Mn(II), presumably due to the higher reduction potential of Mn(III), and to its ability to induce oxidative stress (Ali et al., 1985; Archibald and Tyree, 1987; Chen et al., 2001; Reaney et al., 2002; Reaney and Smith, 2005). In rats administered the same doses of Mn(II) or Mn(III) intraperitoneally, brain and blood concentrations were higher in the rats treated with Mn(III) (Reaney et al., 2006).
8.4 Excretion
Manganese is largely removed from the blood by the liver and then excreted through the bile into the intestine (Miller et al., 1967). From the intestine, a large fraction of the biliary manganese is then excreted via feces, while a small fraction is reabsorbed (Schroeder et al., 1966; Davis et al., 1993).
Whole-body clearance half-time is approximately 4 days for the rapid phase and 39 days for the slow phase, during which about two-thirds of the elimination occurs (WHO, 1981). Whole-body retention half-times have been estimated to be 13 to 37 days in humans who ingested tracer levels of radioactive manganese (as manganese chloride) (Mena et al., 1969; Sandstrom et al., 1986; Davidsson et al., 1989a). Half-lives in the brain have been reported to range from 50–220 days in rats and non-human primates (Takeda et al., 1995; Health Canada, 2010). Although absorption by the brain is mediated by facilitated transport, efflux from the brain has been proposed to occur at a slower rate through diffusion, thus allowing a potential for accumulation over time (Yokel and Crossgrove, 2004).
Direct transport across the intestinal wall from serum can contribute to excretion in the feces (Bertinchamps et al., 1965; Garcia-Aranda et al., 1984). Small amounts of manganese are excreted in urine, sweat, milk, hair and nails (Merian et al., 2004; Health Canada, 2010). The concentration of manganese in urine is generally low (Alessio et al., 1989; Minoia et al., 1990; Park et al., 2003) and urinary excretion is considered to be a poor biomarker of body burden from oral and inhalation exposure (Andersen et al., 1999). Hair has also been used as a biomarker of environmental manganese exposure and body burden in a number of studies, though proper cleaning is required to ensure removal of exogenous manganese contamination so as to examine only metabolically incorporated manganese (Eastman et al., 2013).
Infants, and especially neonates, have a reduced capacity for biliary excretion compared to adults (Cotzias et al., 1976). As a result, neonates and young children will acquire a higher body burden of manganese from a given exposure compared to adults, which along with the important neurodevelopmental processes occurring in neonates, renders them particularly susceptible to manganese-induced toxicity (Neal and Guilarte, 2013).
8.5 PBPK models
Physiologically-based pharmacokinetic (PBPK) models for manganese have been developed for rats (Teeguarden et al., 2007a; 2007b; 2007c; Na et al., 2008 ; Nong et al., 2008; 2009; Yoon et al., 2009 a; 2009b), monkeys (Nong et al., 2009; Schroeter et al., 2011; 2012) and humans (Schroeter et al., 2011; Yoon et al., 2011). The PBPK models published from 2008 include both the oral and inhalation routes. Injection routes (i.p., s.c. and i.v.) were also included in human and monkey models (Schroeter et al., 2011) to allow the evaluation of experimental data obtained with radiolabeled manganese (54Mn). Lactational transfer in rats (Yoon et al., 2009a), as well as fetal dose and disposition of manganese in rats (Yoon et al., 2009b) and humans (Yoon et al., 2011) (mother and fetus) can be estimated. A PBPK model was also developed for transport of inhaled manganese from the olfactory mucosa to the striatum in rats (Leavens et al., 2007). An overview of these models is available in Taylor et al. (2012).
The most recent models for monkeys and humans (Schroeter et al., 2011; 2012) allow estimation of manganese concentrations in target brain tissue (globus pallidus). These models can estimate manganese tissue concentrations following exposure through ingestion, inhalation and injection (i.v., i.p., s.c.). Briefly, they include compartments for liver, lung, nasal cavity, bone, blood, olfactory bulbFootnote 1, cerebellumFootnote 2, globus pallidusFootnote 2, pituitary glandFootnote 2, and remaining body tissues. The models include saturable manganese tissue-binding capacities, preferential fluxes of manganese in specific (brain) tissues and homeostatic control processes (i.e., reduced intestinal absorption and induced biliary secretion at elevated levels of exposure).
Both the human and the monkey models were validated against available manganese tracer data. The monkey model validation included fecal excretion (after s.c. injection or inhalation), whole-body retention (after i.p. injection, i.v. injection or oral exposure) and brain tissue concentrations (following inhalation exposure). The human model was able to capture the dose-dependent characteristics of manganese disposition (whole-body retention, plasma retention) across multiple exposure routes. The authors suggest that the models used to estimate exposure concentrations that would result in increased tissue concentrations could also be applied to a risk assessment based on dosimetry (Shroeter et al., 2011). Although the model can be used to estimate manganese concentrations in brain tissue, such simulations have not been validated in humans.
9.0 Health effects in humans
Numerous epidemiological studies have investigated the effect of manganese exposure on human health. High exposure to Mn, possibly occurring in occupational settings, can cause a clinical neurological disease referred to as manganism, which is characterized by generalized cognitive and motor disturbances, including bradykinesia, widespread rigidity, gait disturbances, falling, dystonia, difficulty walking backwards, and speech difficulties. In addition, many studies have shown that lower exposures to Mn, particularly by inhalation, can result in deficits in neurofunctional outcomes such as impaired fine motor skills, eye–hand coordination and reaction time. Moreover, some recent epidemiology studies have suggested an association between exposure to manganese in drinking water and neurological effects in children (Bouchard et al., 2011; Khan et al., 2011; Roehls et al., 2012; Oulhote et al., 2014).
9.1 Essentiality
Manganese is considered to be an essential element by Health Canada and other international agencies (NRC, 1989; IOM, 2001; Expert Group on Vitamins and Minerals, 2002; National Institutes of Health, 2011; WHO, 2011). Manganese is an essential cofactor for some enzymes (pyruvate carboxylase, Mn-superoxide dismutase) (NRC, 1989; IOM, 2001). In addition, manganese can serve as a cofactor to activate enzymes (decarboxylases, hydrolases, kinases, etc.), that are also activated by other similar divalent cations, and not be altered by manganese deficiency (EVM, 2002). The enzymes requiring manganese have multiple functions, including antioxidant defence, bone formation, and the metabolism of carbohydrates, cholesterol, and proteins, in addition to utilization of other nutrients including the vitamins biotin and ascorbic acid (Saudin et al., 1988; IOM, 2001).
Mn deficiency is extremely rare and symptoms are not well defined (Dupont and Tanaka, 1985; IOM, 2001). In animals, bone and cartilage malformations, impaired growth, reproductive functions, neurologic disturbances and metabolism of glucose, carbohydrates (diabetic-like symptoms) and lipids are observed (EVM, 2002). In humans, skin anomalies such as erythematous rash, slow growth of nails, reduced bone density, hair depigmentation, and hypocholesterolemia are observed with diets that have very low levels of manganese (approximately 0.01–0.34 mg/day).
The IOM established Dietary Reference intakes for manganese, which were adopted by the Health Products and Food Branch of Health Canada. No Estimated Average Requirement (EAR) was established because of the lack of sufficient data on dose and response. Adequate Intake levels (AI) were calculated based on dietary data from average population intakes among healthy individuals. The established AIs are 0.003 mg/day for infants 0–6 months, 0.6 mg/day for infants 6–12 months, 1.2 mg/day for children 1–3 years old, 1.5 mg/day for children 4–8 years old, 2.3 mg/day for adult males, 1.8 mg/day for adult females, 2.0 mg per day for pregnant females and 2.6 mg/day for breastfeeding females, based on median intake data from the Food and Drug Administration Total Diet Study for children, adolescents and adults, and on human milk concentrations for infants (IOM 2001; Health Canada 2007b).
Tolerable Upper Intake Levels (UL) were set at 2–6 mg/day for children 1–18 years old, 11 mg/day for adults, and 9–11 mg/day for pregnant and lactating women (IOM, 2001). No UL was determined for infants, since toxicity data are insufficient, and it is not clear at what age infants can develop mechanisms to maintain manganese homeostasis (IOM, 2001). The American Academy of Pediatrics’ fifth edition of the “Pediatric Nutrition Handbook” and the American Society for Parenteral and Enteral Nutrition report both recommend 1 µg Mn/kg bw per day for parenterally-fed neonates (3–10 kg), though retention of intravenously administered manganese is close to 100% (compared with 8% from breast milk ingestion) (Committee on Nutrition, 2003; Mirtallo et al., 2004; Aschner and Aschner, 2005).
The UL for adults is based on the absence of observed effects in healthy adults consuming a Western-type diet containing up to 10.9 mg/day of manganese (NOAEL of 11 mg/day indicated in Greger, 1999), and in a study with manganese-rich, vegetarian diet, possibly containing 13 to 20 mg/day of manganese (Schroeder et al., 1966). A LOAEL of 15 mg/day was identified in Davis and Greger (1992). An increase in lymphocyte manganese-dependent superoxide dismutase activity was observed after 90 days of supplementation at this dose. No dietary study that assessed the neurological effects of manganese in humans at the UL level was found.
The European Commission’s Scientific Committee on Food set a maximum manganese concentration of 100 μg/100 kcal for infant formula (SCF 2003). The derivation of this value is not clearly explained, but the commission identified manganese’s neurotoxicity as a topic of growing concern.
Manganese deficiency is not expected in Canada, as food is the main source of intake, and the Canadian TDS (Health Canada, 2009) shows that the population is meeting the AIs established by the IOM. Natural health products (i.e., via supplementation with manganese) can also contribute significantly to the daily intake, up to 9 mg/day in Canada.
9.2 Acute toxicity
Accidental ingestion of low doses of potassium permanganate (about 1.8 mg Mn/kg bw/day) for 4 weeks in a 66-year-old man was associated with muscle weaknesses and neurological disturbances such as impaired mental capacity (Holzgraefe et al., 1986). However, because of his exposure to other substances, it is unclear whether the effects were caused by manganese. Another case study reported a 50-year-old man being lethargic, vomiting, and suffering diffused abdominal pain, profuse diarrhea, liver failure, acute renal injury, acute respiratory distress, myocardial dysfunction, and shock with lactic acidosis after ingesting hydrated manganese sulfate (three table spoons daily, total duration unknown). Death occurred within 72 hours. It should be noted that the man was on a protein-free diet and consuming several herbal teas during a liver-cleansing diet (Sanchez et al., 2012).
9.3 Epidemiology
Chronic exposure to air-borne manganese has been repeatedly associated with adverse neurological effects (Health Canada, 2010). Cohort, cross-sectional and case-report studies have been conducted on the effects of high air-borne manganese exposures in welders and workers plus populations living in the surroundings of ferroalloy plants (Roels et al., 1992; Lucchini et al., 1999; Mergler et al., 1999; Bowler et al., 2006; Bouchard et al., 2007b; Lees-Haley et al., 2007; Myers et al., 2009; Riojas-Rodríguez et al., 2010; Criswell et al., 2011; Menezes-Filho et al., 2011; Meyer-Baron et al., 2013; Michalke and Fernsebner, 2014; Yoon and Ahn, 2015).
The relationship between occupational exposure to manganese and subclinical neuropsychological tests are equivocal, but some alterations in visual reaction time, eye–hand coordination, motor performance tests or hand steadiness have generally been found. Manganism was observed in the most highly exposed groups, with symptoms such as bradykinesia, speech difficulties, gait disturbances, widespread rigidity, dystonia, propensity to fall backward, and a characteristic way of walking on the toes with the spine erected and elbows flexed. It is not clear whether the effects associated with exposure via inhalation can be induced via the oral route. Extrapolation across routes is difficult because of the toxicokinetic differences observed across routes of exposure and oxidation states (Aschner et al., 2005; Roels et al., 2012).
Many cross-sectional epidemiology studies have observed associations between higher exposure to manganese and neurological outcomes in infants and children, such as behavioral disinhibition, and lower scores in tests of executive function, reading and digit agility tests (Kawamura et al., 1941; Kondakis et al., 1989; He et al., 1994; Woolf et al., 2002; Wright et al., 2006; Ericson et al., 2007; Wasserman et al., 2011; Khan et al., 2012; Bhang et al., 2013; Chen et al., 2014; Yu et al., 2014; Haynes et al., 2015).
A recent cross-sectional epidemiology study conducted in Quebec investigated the association of manganese in the drinking water supply with intellectual impairment of school-aged children (Bouchard et al., 2011). This study included 362 children between the ages of 6–13 years who lived in communities supplied by groundwater containing manganese at various levels (range from 1 µg/L – 2700 µg/L, with a median of 34 µg/L), as measured in home tap water (MnW). The authors measured the concentration of manganese in the children’s hair (MnH), in addition to their performance on the Wechsler Abbreviated Scale of Intelligence (IQ). A 6.2 point difference in IQ scores was reported between children in the lowest (median level of 1 µg/L) and highest (median level of 216 µg/L) quintiles of exposure to MnW. Higher MnW was associated with lower full scale and performance IQ scores after adjusting for covariates (i.e., maternal education and nonverbal intelligence, family income, home stimulation score, and family structure). Higher MnH was associated with lower full scale IQ score, after adjusting for the same covariates. MnH increased with water consumption of manganese, but not with the dietary manganese (semi-quantitative food frequency questionnaire), suggesting a difference in bioavailability between the forms of manganese in water versus food.
In a second publication from this group, an inverse relationship was also reported between neuro-behavioral functions (memory, attention, motor function, hyperactivity) and manganese exposure in the same children (n = 375) (Oulhote et al., 2014). The authors reported an inverse association between standardized (log10-transformed) MnW or MnH and memory, attention or motor function, after adjusting for confounders (i.e., child’s sex, age, maternal education, nonverbal maternal intelligence, family income, maternal depression, and water lead concentrations). For hair Mn, one standard deviation (SD) increase in log10 MnH was associated with a 24% decrease in the standard deviation of memory scores (95% CI: –36, –12%) and a 25% decrease in attention SD scores (95% CI: –41, –9%), after adjusting for confounders. No significant association was observed between MnH and motor function. For water Mn, one SD increase in log10 MnW (corresponding range not provided by the authors) was associated with a 14% decrease in memory function SD scores (95% CI: –24, –4%), and a weak negative association with motor function (decrease of 11% in SD scores, 95% CI: –21, –0.4%). Steeper decline in memory and motor function were reported at water manganese concentrations above 100 and 180 µg/L, respectively. The association between MnW and hyperactivity or attention was not significant.
Although the non-metal contaminants in drinking water were not assessed, these studies did consider several covariates (e.g., lead and arsenic) in the drinking water, socio-economic status, and maternal factors that may confound the association between manganese and cognitive abilities. However, the risk of bias in these studies cannot be discarded. Few details on eligibility criteria of subjects and characteristics of lost-to-follow-ups were provided. Misclassification of the exposure was possible for different reasons: MnW was only measured once, residing in one’s current home for three months was sufficient for inclusion (previous exposures to manganese not considered), hair as a biomarker can bias exposure, there were no information on diet or soil concentration included, and no information was provided on the timing and duration of exposure levels during critical periods of development. Blinding of the investigators to the exposure levels of participants was not discussed. Finally, the confidence intervals were large and borderline significance was reported for many effects observed.
Children aged 7–9 years (n = 404) were enrolled in the cross-sectional Communities Actively Researching Exposure Study in Ohio from October 2008 to March 2013 (Haynes et al., 2015). Covariates such as blood and hair manganese levels, as well as the outcome variables (cognitive development via the WISC-IV) were measured during the first visit. Both high blood (> 11.2 μg/L) and high hair (> 747 ng/g) manganese concentrations were significantly associated with lower full-scale IQ scores, compared with the control groups (blood: 8.2–11.2 μg/L; hair: 207–747 ng/g), after adjusting for confounders (the most important being creatinine, blood lead, community, gender, and parent’s IQ and education). The authors only looked at the association between the predictors and the outcomes, and did not interpret their results in terms of risk. The measured associations can be biased since the information on participants and exposure was poorly detailed. For example, the authors excluded participants with missing data for any model covariates and those with higher manganese levels, which could bias the observed relationship (e.g., if participants with missing data had low manganese and low IQ, or high manganese and high IQ, then excluding these would bias the results away from the null in both scenarios, artificially inflating the effects). The characteristics of the excluded participants were not presented, so it is unknown how well the included subjects represent the population. Exposure was subjected to misclassification, since previous exposures and variations over time were poorly assessed (i.e., hair and blood are limited bioindicators; there were no estimate of the manganese total intake or the contribution from drinking water; only one measurement of the manganese bioindicators and of the other covariates levels was taken). There was also a risk of confounding, since the baseline characteristics were not stratified according to exposure groups (cannot assess differences other than manganese at baseline) and the statistical models were not built in a way to ensure inclusion of all important confounders (changes in the outcome variable should be the basis for covariates selection, and not in the exposure variable). Moreover, manganese was correlated with other included covariates, lowering the confidence in the model. Also, participants could be classified in different quartiles of exposure, depending on the bioindicator chosen as the main predictor variable (hair or blood Mn). There was also a risk of chance finding, and it would have been useful to provide the significance (p-values) of the multivariable models since the results were imprecise (large 95% CI almost including the null).
Several cross-sectional studies conducted in Bangladesh reported associations between manganese levels in drinking water and cognitive performance in school-aged children. Wasserman et al. (2011) investigated the association of manganese and arsenic exposure with intellectual function in 304 children aged 8–11 years old using adapted subtests from the Wechsler Intelligence Scale for Children IV. The authors classified children based on whether well water manganese concentrations were above or below 500 µg/L, and above or below 10 µg/L for As. After adjustments were made (maternal education and intelligence, house type, family ownership of a television, and child height and head circumference), increasing blood manganese concentration (MnB) was associated with a reduction in working memory and perpetual reasoning scores. Weaknesses of this study include the fact that the study was originally designed for another objective and other contaminants of drinking water could be responsible for the observed effects. Also, no account for other sources of manganese was made, and there were no attempts to verify an association between MnW and MnB. Also, the neurological outcomes were measured with an adapted scale which was not validated or standardized. In addition, the lack of quantitation of the exposure quartiles used in the study does not allow for the use of the data in a quantitative risk assessment. Finally, only standard errors of the regression coefficients were presented.
The limitations of the Wasserman et al. (2011) study also apply to an earlier publication by the same authors (Wasserman et al., 2006). In the earlier study, there was a dose-related reduction in full-scale, performance, and verbal raw scores after adjustment for confounders (the same as in the 2011 study). In contrast with the 2011 study (where the association with MnB was significant), only the association with MnW was significant in the 2006 study.
Khan et al. (2012) examined the associations between manganese and arsenic exposure from drinking water and the academic achievement of 840 children in Bangladesh. Intellectual function was evaluated in language and mathematics. A significant association between MnW > 400 µg/L and decreased mathematics test scores was observed after adjustment for confounders (i.e., arsenic, school grade, maternal education, paternal education, head circumference, and within-teacher correlations in rating the children). In a previous study by the same authors and with a similar protocol, increasing MnW was associated with classroom negative behaviors after adjustment for confounders (i.e., arsenic, sex, BMI, maternal education, and arm circumference) (Khan et al., 2011). The conclusions of these two studies may be qualified by the lack of account of other possible exposure to other neurotoxic substances (e.g., incomplete arsenic and lead adjustments for exposure), the lack of a thorough characterization of the exposure (and consideration of other sources of manganese such as air and dust), the high variability of the results (wide 95% CI), and the risk of teacher’s bias.
The association between cord blood manganese levels and psychomotor sub-scales was assessed in a cohort study of socially advantaged individuals (n = 247 pregnant women and their offspring) in Paris (Takser et al., 2003). The children were assessed during three psychological examinations (at 9 months, 3 years, and 6 years of age). Attention, non-verbal memory, and hand skills were negatively associated with exposure, after adjustment for confounders (child’s gender, mother’s educational level). However, there were no relationships between birth exposure to manganese and the general psychomotor indices, Brunet–Lézine developmental quotient at 9 months or McCarthy general cognitive index (GCI) at 3 and 6 years. The contribution of manganese exposure from drinking water was not clear. The authors did not mention a method to factor the intra-individual correlation (repeated measures on the same individual through time) into the statistical analysis increasing the risk of type I error. Although the authors indicated all regression models were adjusted for maternal and cord blood lead levels, different sets of covariates were used and it was not clear which ones were chosen for each model.
A longitudinal multicentre cohort study in Shanghai, China investigated the effects of prenatal exposure to manganese at environmental levels through examination of umbilical serum concentrations of manganese (MnUmbi) and administration of Neonatal Behavioural Neurological Assessments (NBNA) (Yu et al., 2014). The authors examined 933 mother–newborn pairs by measuring MnUmbi and evaluating corresponding newborns using the NBNA test to assess the behavioral development of newborns (reflexes, functional abilities and behavioral status). Being in the highest quartile of MnUmbi was associated with lower NBNA score (statistical significance of the regression coefficient) after adjusting for confounders (parents’ age, education, incomes, occupation, and smoking status, gestational age, gender, birth weight, lead, and mercury) . However, the low rate of participation (70% of recruited subjects) could have induced selection bias. Maternal serum during pregnancy was not analyzed to assess a possible relationship with intrauterine development. There was no long-term follow-up for impact on childhood development. No adjustment for important confounders (other exposure sources, environmental risk factors, metals exposure) was conducted. Moreover, residual confounding is likely since there were large differences in socio-economic status between exposure groups. The authors did not explain the clinical significance of the changes (importance of a 1.1 decrease in the regression coefficient), and it was not clear why the relationship was described as non-linear since there was no clear dose-response effect. The included covariates were likely to be collinear (e.g., maternal education and occupation), but the authors did not present a rationale for including these together in the model.
The association between environmental contaminants exposure and neurodevelopment at 2 years old was investigated with the Taiwan Birth Panel Study (n = 230 pairs of non-smoking mothers and their children) (Lin et al., 2013). Exposure in utero to manganese levels above the 75th percentile (i.e., 59.3 µg/L) in the umbilical cord blood was associated with lower cognitive and language quotients of the Comprehensive Developmental Inventory for Infants and Toddlers compared with levels below the 75th percentile (statistical significance of the regression coefficient), after adjusting for maternal age, sex, smoking, home support and stimulation, and arsenic and mercury levels in cord blood. Global, motor, social, and self-help quotients were not associated with the model. No rationale was provided for including less than 50% of the selected participants, which differed from the general population by having mothers with higher levels of education, umbilical cord blood with greater levels of mercury and lead, and higher birth weight. Moreover, only one measurement of the contaminants (lead, mercury, manganese, arsenic) was done. Finally, the study focussed on co-exposure effects, and the authors did not indicate whether they adjusted for lead in the manganese model.
Case studies in two children (6- and 10-year old girls) drinking well water containing high levels of manganese (up to 2.4 mg/L in both cases) were reported by Sahni et al. (2007) and by Brna et al. (2011). High serum levels of manganese and neurological symptoms such as pica, behavioral changes, speech difficulties, lack of coordination, difficulty walking, and social withdrawal were observed in both girls. The rest of the family members were asymptomatic in each situation. The authors have suggested that a metabolic disorder involving divalent metals could have been associated with the development of the symptoms.
A case study of a 10-year-old Massachusetts boy who for 5 years had been drinking turbid well water containing manganese at a concentration of 1210 µg/L was reported by Woolf et al. (2002). The child had normal cognition, neurology, fine motor skills, balance, classroom performance, magnetic resonance imaging, and IQ. However, his performance as reflected in his ranking in the general memory index (17th percentile), verbal memory (19th percentile), visual memory (14th percentile), and learning index (19th percentile) were below average. His teachers noticed a lack of focus in the classroom. Causality is uncertain, since the water contained high levels of iron (1570 µg/L) plus toxic wastes had been an issue of concern by residents in their highly industrialized neighbourhood. Only one water sample was collected at the end of the 5-year exposure.
Some cross-sectional studies (Zota et al., 2009; Chen et al., 2014; Eum et al., 2014; Guan et al., 2014) and one cohort study (Yu et al., 2013) investigated the association between manganese exposure and body weight at birth. A minority of these observed an association between manganese and reduced body weight (those conducted in Asia with a cross-sectional design). Each of the studies with a positive association suffered from several limitations, such as designs not allowing determination of temporality (direction of effect), selection bias, and lack of adjustments for important covariates that limits establishing the causality of manganese for the observed health effects.
On the other hand, other studies did not observe an association between high environmental exposure to manganese and neurological outcomes (Vieregge et al., 1995; Torrente et al., 2005a; Hernandez-Bonilla et al., 2011). The exploratory study by Torrente et al. (2005a) found no correlation between the concentration of different elements in hair (average manganese 0.18–0.26 µg/g) and the cognitive status of children 12–14 years old (n ± 100) living close to industrial areas in Catalonia, Spain. Also, the cross-sectional study by Vieregge et al. (1995) found no difference in neurological impairment item values or in Parkinson disease scores between two cohorts of adults above 40 years old (homogenous in terms of age (mean of 57 years old), gender, nutrition, and drugs) exposed to different levels of manganese in well drinking-water for at least 10 years in rural Germany (n of group A with manganese < 50 µg/L = 74; n of group B with manganese > 300 µg/L = 41). Blood manganese levels were not statistically different between the two groups. The cross-sectional study by Hernandez-Bonilla et al. (2011) found no significant difference in neuromotor outcomes between children 7 and 11 years old residing in rural communities with high or low manganese biomarkers of exposure (blood: 9.7 vs 8.2 µg/L; hair: 12 vs 0.57 µg/g), after adjusting for covariates (blood Pb, Hg, sex, age, maternal education), except for a weak association between finger tapping tests and manganese in blood only. The results generally showed a lack of association and were not interpreted in terms of risk by the authors. The marginal association with finger tapping ability is not conclusive since the authors did not adjust for other covariates (smoking by the parents, differences in nutrition, and presence of 13 overweight children in the low exposure group compared with one in the high exposure group, possibly indicative of other socio-economical factors); also, past exposures was not assessed. Conclusions based on these three studies showing no effects are limited because of their cross-sectional design, the possibility that only healthy individuals stayed in areas with higher concentration of the element (sick survivor effect), the possibility of other sources of exposure or unaccounted variations in the past, and the absence of adjustment for major covariates (e.g., nutrition status).
Although manganese is essential to human health, case reports, cross-sectional studies and cohort studies support an association between exposure to manganese in drinking water at elevated levels and neuropsychological issues in infants and children, such as changes in behavior, lower IQ, speech and memory difficulties, lack of coordination and movement control. Cross-sectional and case report studies reported associations between neuropsychological outcomes and high levels of manganese (hundreds of µg/L) in drinking water; meanwhile, cohort studies reported these associations with prenatal manganese levels as reflected in cord blood manganese. Most studies did not allow determination of temporality (i.e., effect coming after the cause) of the association. The risk for spurious associations was estimated to be high, and exposure measurements were generally poor and relied on a single measurement of a single sample. A better understanding of the manganese neurotoxicity mode of action would allow the identification of specific neurological biomarkers of effect and help measure early signs of toxicity.
Manganism has been observed following inhalation of manganese in occupational settings; however, it is not clear whether this effect can be extrapolated to the oral exposure route because major toxicokinetic differences exist between inhalation versus oral intake and developmental versus adult sensitivity. More reliable exposure measurements that identify a clear toxicological point of departure for the neurological effects are needed in order to conduct a dose-response analysis for the endpoints monitored. It should be noted, however, that the epidemiology studies qualitatively support neurotoxicity as a potential critical endpoint.
10.0 Health effects on experimental animals
Manganese is readily taken up into the central nervous system (CNS), although uptake into a wide range of other tissues including the lungs, kidneys and testes has also been reported. A number of studies in animal models have demonstrated the CNS to be the most susceptible target of toxicity resulting from oral exposure to manganese, followed by reproductive systems. The following sections describe toxicological information based on oral exposure to soluble manganese salts.
It should be noted that animal studies using manganese salts often do not clearly indicate whether the reported dose is reflective of manganese or a manganese complex (e.g., MnCl2, MnCl2·4H2O). Health Canada has therefore reported the dose as worded by the authors of the study. Where possible, authors of studies considered possibly key to the assessment have been contacted to confirm the dosing has been correctly interpreted. In general, the reported dosing in the majority of studies has been found to refer to elemental Mn.
10.1 Acute toxicity
The acute oral lethality of manganese varies with chemical species as well as with the route of exposure (gavage vs dietary ingestion). Manganese compounds appeared to exhibit lower acute toxicity when provided in feed than when provided by gavage (Finley and Johnson, 1996; U.S. EPA, 2003; Health Canada, 2010). The median oral lethal doses (LD50 values) ranged from approximately 250 mg Mn/kg bw (as manganese chloride) to 1082 mg Mn/kg bw (as manganese acetate) in adult rats exposed by gavage (Hazaradze, 1961; Smyth et al., 1969; Kostial et al., 1989; WHO, 2011). In contrast, repeated exposure to a similar dose of manganese (50,000 ppm) as manganese sulfate in feed resulted in no death in rats exposed for 14 days (NTP, 1993).
Following a single exposure of rats to aqueous MnCl2 (50 mg/kg MnCl2·H2O) by gavage, a number of neurological effects were reported, including a significant and reversible decrease in total activity, along with delayed acquisition of an avoidance reaction in response to unconditioned and conditioned stimuli, an increased latent period of conditioned reflex activity, and a temporary worsening of the learning process (Shukakidze et al., 2003).
10.2 Short-term and chronic exposure
10.2.1 Neurotoxicity
A number of studies have identified neurotoxicity as a particularly sensitive endpoint following exposure to manganese. Neurotoxic effects resulting from exposure to manganese can be categorized as affecting behavioral endpoints (including information related to altered reflex, motor activity, learning, memory, or sensory alterations), structural endpoints (including gliosis and neuroinflammation, in addition to neurostructural alterations), and neurochemical endpoints (altered neurotransmitter systems). Neurotoxic effects resulting from exposure to manganese both during development and in adulthood are presented in this section.
10.2.1.1 Neurobehavioral endpoints in neonates and juveniles
Neurobehavioral effects were reported in rats and mice (pups or neonates) following exposure during gestation, during lactation and/or after weaning through direct exposure (diet, drinking water or gavage). Similarly, neurobehavioral effects have also been reported in non-human primates exposed to manganese through formula.
A transient increase of locomotor activity was observed in mice exposed to manganese on PND 2–24 through lactation (dams exposed to 5 mg Mn/mL (equivalent to 5000 ppm Mn) in drinking water) and on PND 25–180 (3 µg/mL or 3 ppm manganese in drinking water). The actual dose of exposure (in mg/kg bw to the dams) was not provided, and exposure to the pups during lactation is unknown. Locomotor activity (measured at 30-day intervals) was increased at PND60 and PND90 only, and not on PND 30, 120, 150 or 180 (Chandra et al., 1979a). The effect of manganese on motion at higher doses was observed in a three-generation study in mice: abnormal motion was reported in the gait of 2 neonates of the 3rd generation litter exposed to 25 mg Mn/kg bw per day (Ishizuka et al., 1991). Similarly, increased spontaneous motor activity was observed in neonatal rats exposed orally (per os) to an aqueous solution of manganese chloride at the highest dose tested (50 mg MnCl2/kg bw per day) for 50 days (PND1 to PND49) (Brenneman et al., 1999). Effects were not reported at the 25 mg MnCl2/kg bw per day dose level.
Alterations in open field activity (assessment of behavior and locomotion) were reported in male mice exposed during the juvenile period only or during both the juvenile (PND20 to PND34) and adult periods (PNW12 to PNW20) to 10 or 30 mg Mn/kg bw per day as manganese chloride in saline for 2 weeks via gavage. It was found that developing mice were more sensitive to the effects of Mn, and that early exposure increased susceptibility to manganese exposure when the treated animas were adults. No effects were observed in female mice (Moreno et al., 2009b). NOAEL of 250 µg Mn/day was reported following administration in a 10% sucrose solution by micropipette (LOAEL: 750 µg Mn/day) for neurobehavioral effects (negative geotaxis task and traveled scores of rats challenged with cocaine). Rats were exposed for 21 days (PND1 to PND21; Reichel et al., 2006).
Manganese exposure is associated with various sensory effects in young animals. Effect on orientation was assessed by the air righting reflex in rats after in utero and lactational exposure (from GD10 to PND21), via maternal dietary exposure to 32, 160, or 800 ppm MnCl2•4H2O. Male offspring exposed to a maternal dose ≥ 160 ppm and female offspring exposed to 800 ppm exhibited significant decrease in air righting indices (Ohishi et al., 2012).
Decreased olfactory discrimination was reported in rats exposed orally to 500 µg Mn per day as MnCl2 in a 10% sucrose solution from PND1 to PND20, and trends toward decreased memory and learning ability were observed, as evidenced by burrowing detour and passive avoidance tests (Tran et al., 2002a; Tran et al., 2002b). In neonatal rats orally exposed (PND1 to PND21) to 25 or 50 mg MnCl2/kg bw per day given as MnCl2.4H2O via micropipette for 21 days, an increased pulse-elicited acoustic startle response amplitude was observed (Dorman et al., 2000) (it is presumed that the actual dose reported by the authors refers to Mn).
The neurobehavioral effects resulting from an excess of manganese in soy formula were assessed in infant rhesus monkeys fed a commercial cow's milk–based formula (Cow; 50 μg Mn/L), a commercial soy protein–based formula (Soy; 300 μg Mn/L) or a soy formula with added manganese (Soy+Mn; 1000 μg Mn/L) for 4 months following birth (Golub et al.,2005). Corresponding doses reported by the authors were 0.018, 0.106, and 0.323 mg Mn/kg bw per day, respectively (as noted in an erratum:Golub, 2012). An extensive behavioral test battery (motor, cognitive and social domains, as well as specific tests related to the dopamine system)Footnote 3 was administered over an 18-month period (approximately equivalent to 6 years of age in children). It is important to note that besides differing in manganese content, the cow’s milk–based and soy-based formulas differ in several nutritional dimensions related to protein, carbohydrate and fat composition, and other components that could contribute to differences in behavioral responses reported (Golub et al., 2005). Thus, the Cow group does not represent an appropriate control to assess the effect of manganese in the Soy and Soy+Mn groups for quantitative risk assessment purposes. The effect of manganese would be best assessed by comparing the Soy+Mn group to the Soy group; however, statistical analysis to compare these two groups was not provided. Nevertheless, changes in activity/sleep patterns and in social interactions (less play behavior and more affiliative clinging in social dyadic interactions) were reported in monkeys of the Soy and Soy+Mn groups when compared to the Cow group. The Soy and Soy+Mn groups also exhibited shorter wake cycles and shorter period of daytime inactivity than the Cow group, with no apparent differences between the Soy and the Soy+Mn groups (statistical analysis not available). All three groups did not differ significantly in dopamine and serotonin metabolite concentrations (HVA and 5HIAA) in the cerebrospinal fluid (CSF), stereotypy and continuous performance tests. This study may indicate a sensitivity of social behavior and DA systems to early dietary manganese exposure; however, it should be noted that nutritional differences between Cow and Soy formula could contribute to differences in behavioral responses. Overall, results indicated no group differences in the more highly structured testing situationsFootnote 4 used to assess specific cognitive functions including learning, memory and attention. However, poor engagement of the infants with the formal tests of cognitive function was seen at the ages tested, and additional testing at more mature ages may provide further insight (Golub et al.,2005; Golub, 2012).
The effect of postnatal exposure to manganese on both non-associative and associative behaviors was also investigated in neonatal rats given oral supplements of manganese (750 μg/day) on PND 1–21 in a study by McDougall et al. (2008) (see also the Neurochemical Endpoint Section for results on the presynaptic dopamine function). The effects of postnatal manganese exposure on nigrostriatal functioning were evaluated by assessing rotorod performance and amphetamine-induced stereotypy in adulthood. Manganese had no impact on the rotorod performance; however, manganese-exposed rats exhibited substantially more amphetamine-induced stereotypy than vehicle controls. In terms of associative processes, manganese exposure did not alter performance on any aspect of the cocaine-induced conditioned place preference (CPP) task (preference, extinction, or reinstatement testing), nor did manganese affect progressive ratio responding (sucrose-reinforced operant responding, a measure of motivation). However, acquisition of a fixed ratio task was impaired, suggesting a deficit in procedural learning.
Other behavioral effects reported in neonate rats were observed at higher oral exposures (estimated at 8.8 mg Mn/kg bw per day (from 500 µg/d) and above), and include decreased olfactory discrimination (Tran et al., 2002a). In addition, impaired neurobehavioral endpoints reflecting increased anxiety and alterations of other emotional behavior, decreased learning and memory ability, increased signs of hypo/hyperactivity and altered motor activity (Kristensson et al., 1986; Pappas et al., 1997; Brenneman et al., 1999; Liu et al., 2006 ; McDougall et al., 2008 ; Kern et al., 2010 ; Kern and Smith, 2011; Molina et al., 2011 ; Nowak et al., 2011;Peneder et al., 2011; Betharia and Maher, 2012; Ohishi et al., 2012; Beaudin et al., 2013) were reported at levels ≥ 25 mg/kg bw per day.
A detailed investigation of the neurodevelopmental effects of manganese exposure in rats was provided by Kern et al., 2010, who exposed neonatal Sprague-Dawley rats to oral doses of 25 or 50 mg Mn/kg bw per day in sucrose solution via micropipette for 21 days following birth. The study conducted a battery of neurological tests (open arena, elevated plus maze, and 8-arm radial maze) on the rats (n = 15–20 males/treatment) to assess the effects of oral exposure to manganese during a period that corresponds to the development of dopaminergic pathways in regions of the brain that are important in the regulation of executive function behaviours (involving attention, learning, and memory). In addition to the behavioural tests, levels of dopamine receptor and transporter proteins were measured in the brain (described in the “neurochemical endpoints” of this section). Though a NOAEL could not be identified, a LOAEL of 25 mg Mn/kg bw per day can be established for this study, based on increased stereotypic behavior on a greater number of session days during the 8-arm radial maze test (shift in goal-oriented behavior, indicating impaired spatial memory) and a reduced level of D1-like receptors in the dorsal striatum. At the higher tested dose (50 mg Mn/kg bw per day), the authors also observed altered locomotor activity and behaviour disinhibition in the open area test on PND23, altered learning and increased number of errors in the radial maze on PND23, and impaired learning/memory (delay/failure to reach the learning criterion and increased number of learning errors in the 8-arm radial test) over PND 33–46. Manganese exposure (up to 50 mg Mn/kg bw per day) had no apparent impact on fear and anxiety (as measured by elevated plus maze performance) (Kern et al., 2010).
In a subsequent study aiming to document whether early exposure to manganese results in neurobehavioral and neurochemical effects lasting into adulthood, rats exposed orally to the same doses of manganese as in the previous study, also for 21 days post-natally without further exposure, were examined later in life. It was reported that neonatal exposure to manganese led to altered dopamine receptor levels and astrogliosis (as measured by Glial Fibrillary Acidic Protein, or GFAP) in adulthood. Although behavioural disinhibition or altered locomotor activity was not observed in adults, an evaluation of behavioral performance (as measured by activity in the center zone of the open arena) in the absence (PND97) and presence (PND98) of a D-amphetamine challenge revealed that exposed animals exhibited an enhanced locomotor response to the D-amphetamine challenge (Kern and Smith, 2011). Collectively, these results suggest that early exposure to manganese can result in lasting effects on the dopaminergic system.
The same neonatal model and dosing regimen was used by Beaudin et al. (2013) to further explore the chronic effects of manganese exposure on sensori-motor development. The study compared the effects of early life and lifelong exposure to manganese on fine sensorimotor function in male Long-Evans rats. The staircase test was used to objectively measure effects that are relevant to motor outcomes studied in pediatric research. The authors exposed the animals (n = 11/group) orally to 25 mg Mn/kg bw per day or 50 mg Mn/kg bw per day for either a short post-natal period of 21 days or a life-long period (through adulthood). Testing conducted in adulthood showed a deficit in performance (staircase test, taking and eating pellets) at the 25 mg Mn/kg bw per day dose level, and performance was worse in rats exposed to this dose daily over a lifetime than those only exposed for a brief period of 21 days postnatally. These findings support findings in epidemiological work reporting a deficit in fine sensorimotor function in children following exposure to manganese (Beaudin et al., 2013; Zoni and Lucchini, 2013).
10.2.1.2 Neurobehavioural endpoints reported in adults
Neurobehavioral alterations following oral exposure to manganese in adulthood were reported in a number of studies. Rats exposed to manganese via drinking water (1 mg MnCl2•4H2O/mL) exhibited signs of hyperactivity and hypoactivity such as increased/reduced spontaneous motor activity, altered response to D-amphetamine test, shorter distance traveled, and decreased open field activity (Nachtman et al., 1986).
In mature female albino rats treated by gavage with manganese chloride (0.357 and 0.714 mg Mn/kg bw per day, in distilled water) for 15 or 30 days, increases in manganese and cholesterol levels in the brain (hippocampus, cerebellum and cortex) were accompanied by learning impairment (after one training period on day 29, treated rats needed 3.7 times more time to reach the exit of a T-maze compared to controls). The delay in exposed rats was completely corrected by co-administration of mevinolin (an inhibitor of cholesterol biosynthesis) with manganese or by withdrawal of manganese (Öner and Sentürk, 1995; Sentürk and Öner, 1996).
The acquisition of conditioned and unconditioned avoidance reactions and the dynamics of learning and memory impairments were studied in adult rats exposed to manganese before or after training for a multipath maze. Rats were exposed to manganese chloride at doses of 20 or 50 mg MnCl2.4H2O/kg in food for 30 days. Testing was performed immediately after training or 90 days after training. Animals exposed at the higher dose level showed increased aggressivity, frequently fell from the platform in the maze, and were unable to perform the maze tests. Irreversible impairment of the learning process was reported following exposure to manganese; however, manganese did not significantly alter the animals’ ability to reproduce a previously acquired behaviour (completion of passage through the maze) (Shukakidze et al., 2003).
Young male adult Wistar rats (16/dose) were exposed to manganese chloride by gavage (14.84 or 59.36 mg MnCl2•4H2O/kg bw per day, 5 days/week, in distilled water) for 10 weeks, followed by a 12-week recovery period. Statistically significant decreased short- and long-term spatial memory performance in the 8-arm radial maze test and in the spontaneous locomotor activity in the open field activity test were observed at both doses. At post-treatment week 7, locomotor activity returned to normal. Decreased number of acoustic startle responses and their associated prepulse inhibition were also observed.Increased latency of sensory evoked potentials, along with a decrease of their duration, was observed for both doses following 10 weeks of treatment which persisted in the high-dose group only into recovery period. Increased glial fibrillary acidic protein immunoreative (GFAP-IR) density was observed at both doses in the dentate gyrus of the hippocampus but not in the stratum radiatum or the stratum oriens (Vezeret al., 2005). In an earlier study that examined the effect of manganese on locomotor activity, rats chronically exposed to manganese via drinking water (1 mg MnCl2•4H2O/mL) exhibited signs of hyperactivity and hypoactivity such as increased (from weeks 5–7) or reduced (starting week 8) spontaneous motor activity, altered response to D-amphetamine test (increased response from 14–29 weeks that was gone at weeks 41 and 65), shorter distance traveled, and decreased open field activity (Nachtman et al., 1986).
Impaired spatial learning was reported in pregnant Sprague-Dawley rats during the Morris water maze test following a 43-week exposure to an iron-deficient diet (3 mg Fe/kg) that was supplemented with 100 mg Mn/kg, compared to pregnant rats fed control diets (35 mg Fe/kg, 10 mg Mn/kg) (Fitsanakis et al., 2009). In this study, exposure to manganese via diet revealed that iron-deficient status (which occurs more commonly during pregnancy) can increase the effect of manganese on the cognitive system (Fitsanakis et al., 2009).
In male adult rats exposed to 0.1 or 5.0 mg Mn/mL as manganese chloride in drinking water for 8 months, spontaneous motor activity (measured weekly in 60 one-minute-long sessions) was increased at both doses during the first month (Bonilla, 1984a). Further exposure for up to six months did not affect this endpoint, but a significant reduction in motor activity compared to controls was observed at 7 and 8 months. Fine sensory motor development in rats exposed from birth for 21 days and over a lifetime was studied using the staircase test (Beaudin et al., 2013). It was found that exposure to 50 mg Mn/kg bw per day by gavage in early life alone resulted in altered fine motor control in adults. Further, life-long exposed adults displayed additional widespread impairment in reaching and grasping/retrieval performance (Beaudin et al., 2013).
Signs of muscular weakness and rigidity of the lower limbs were reported in four adult male rhesus monkeys (Macaca mulatta) given 25 mg MnCl2•4H2O/kg bw per day orally, corresponding to 6.94 mg Mn/kg bw per dayFootnote 5) for 18 months (Gupta et al., 1980).
Impaired neurobehavioral endpoints were reported in pups, juvenile and/or adult rats and mice exposed to oral doses of manganese (estimated to range from 43.7 to 910 mg Mn/kg bw per day, as manganese chloride), for various periods of time (ranging from 14 days to 21 weeks) via gavage, diet or drinking water (Gray and Laskey, 1980; Ali et al., 1983; Chandra, 1983; Kristensson et al., 1986; Pappas et al., 1997; Calabresi et al., 2001; Torrente et al., 2005b; Liu et al., 2006 ; Vezer et al. 2007; Avila et al., 2008; Molina et al., 2011 ; Nowak et al., 2011; Betharia and Maher, 2012; Fordahl et al., 2012; Shi et al., 2012; Takacs et al., 2012; Krishna et al., 2014). The impaired endpoints observed by researchers included delayed air righting reflex, ataxia, increased learning, memory and locomotor deficits (poorer results in the Morris water maze test, the step-down test), and observation of hypoactivity or hyperactivity, anxiety, negative mood, aggressivity and impulsivity (in the open-field test, the novel object test, the forced swim test, the elevated plus maze test).
10.2.1.3 Structural evaluation of neuronal tissues
Several studies have documented the deleterious effects of manganese exposure on the ultrastructure of brain tissues, such as neuronal degeneration, gliosis (astrogliosis)Footnote 6 or neuroinflammation, in both juvenile and adult rodents.
Alteration of brain tissue was observed at very low dose in juvenile male rats (PND21) exposed to MnCl2•4H2O in water daily (one dose tested, reported as: 50 µg MnCl2•4H2O per rat per day) via gavage for various durations (15, 30, 45 or 60 days, i.e., up to PND80; 6 rats/group). Although only one administered dose was reported, considerable change can be expected in the actual dose on a mg/kg basis, given the changing body weights of the growing young animals throughout the course of the study. The observed alterations include neuronal degeneration in the cerebral cortex of the frontal region and in the cerebellar cortex after 30, 45 and 60 days (no effect at 15 days), neuronal degeneration and neuroglial proliferation in the caudate nucleus after 60 days of exposure, and presence of astrocyte alterations reported to be similar to Alzheimer type-II cells (morphological change) in the area of neuronal degeneration (cerebral cortex) (Chandra and Shukla, 1978).
Marked neuronal degeneration (gliosis, neuronal loss and depigmentation in the region of the substantia nigra) was observed in four adult male rhesus monkeys (Macaca mulatta) given 25 mg MnCl2•4H2O/kg bw per day orally (details on administration not specified) for 18 months, when compared to four control monkeys (Gupta et al., 1980). Other brain regions were not examined.
In male and female rat pups exposed to manganese chloride in utero and through lactation (dams received daily water solution of manganese chloride in the first portion of their feed, leading to estimated doses of 10 or 20 mg MnCl2•4H2O/kg bw per day) for approximately 70 days (15–20 days before pregnancy, during pregnancy, and for one month after parturition with the first portion of food. A marked and dose-dependent gliosis was observed in the offspring at both doses in the caudate nucleus, dorsal and ventral septal nuclei, nucleus accumbens and cerebral cortex (Lazrishvili et al., 2009).
In two studies that exposed young adult rats to daily manganese (20 or 50 mg MnCl2H2O/kg) for 30 days via gavage, changes in the ultrastructure of neurons, interneuron contacts and glial cells were observed in the caudate nucleus, the substantia nigra and the fronto-parietal cortex area. These changes worsened after three months (i.e., after cessation of exposure), resulting in cellular degeneration. Both studies also reported destruction of most of the neurons or astrocytes mitochondria (Bikashvili et al., 2001; Shukakidze et al., 2002). The daily doses tested were 50 mg MnCl2•4H2O/kg (Bikashvili et al., 2001) and 20 or 50 mg MnCl2•4H2O MnCl2/kg bw per day (Shukakidze et al., 2002).
The differential effects of exposure of mice to manganese chloride in saline by gavage (10 or 30 mg Mn/kg bw per day was examined during three life-periods: juvenile (PND 20–34), adult (PNW 12–20) and both juvenile and adult (Moreno et al., 2009a). At ≥ 10 mg Mn/kg bw per day, manganese increased the nitric oxide synthase 2 (NOS2) expressionFootnote 7 in microglia and caused a slight activation of astrocytes (not statistically significant) in juvenile mice, and an increase in astrogliosis was seen in adult mice. The same alterations were observed in mice exposed for both periods, although the magnitude of changes observed was greater, and NOS2 expression was also increased in astrocytes (Moreno et al., 2009a).
Activated astrocytes (as indicated by increased levels of glial fibrillary acidic protein (GFAP) and/or impaired neurogenesis (as demonstrated by increased doublecortin-positive cells and reelin-positive cells, and decreased thyroid-related hormone concentrations) were observed in rats exposed to manganese chloride by micropipette on PND 1–21 (25 or 50 mg Mn/kg bw per day, in a 25% sucrose solution; Kern et al., 2011) or in utero and through lactation (maternal diet concentrations: 32, 160, 800 or 1600 ppm of MnCl2•4H2O) on GD10–PND21 (Ohishi et al., 2012). An increase of GFAP (in the dentate gyrus) was also observed in adult rats following 10 weeks of exposure to manganese via gavage (15 and 59 mg MnCl2•4H2O/kg bw per day in distilled water) (Vezer et al., 2005; Vezer et al., 2007). Preweaning exposure to manganese resulted in increased expression of GFAP in the prefrontal cortex in rats exposed to 25 or 50 mg Mn/kg bw per day (PND 24), and “progressed” to also include increased astrocyte activation in other brain regions that control executive function: the medial striatum and nucleus accumbens at the 50 mg Mn/kg bw per day level (PND 107). These results indicate an effect of manganese exposure on astrogliosis that persisted and/or progressed to other brain regions in adult animals after blood manganese levels had returned to normal (Kern and Smith, 2011).
In adult male mice, increased expression of mRNA GFAP and protein GFAP, heme oxygenase-1 (an oxidative stress marker) and inducible NOS was observed in the substantia nigra following 8 weeks of exposure to 0.4 g Mn/L through drinking water (Krishna et al., 2014).
In utero and lactational exposure (from GD 10 to PND 21) of mice to manganese through the diet (32, 160 or 800 ppm Mn from manganese chloride) resulted in an increased number of reelin-positive cells on PND21 for animals who received 160 ppm manganese and above. Additional neuronal alterations were also observed at the highest dose. The authors proposed that manganese targets immature granule cells and suppresses differentiation into mature granule cells, leading to apoptosis and neuronal mismigration (Wang et al., 2012).
A multigenerational study conducted in mice treated with 25 mg Mn/kg bw per day (as manganese chloride) in drinking water observed cell toxicity in 2 neonatal mice (3rd generation), which was evidenced by abnormal motion in gait. Losses of stainability in granular cells in the external layer of the cerebral cortex, Purkinje cells in the cerebellar cortex, and increases in stainability of the nerve fibers in the cerebellar medulla were reported (Ishizuka et al., 1991).
10.2.1.4 Neurochemical endpoints
An increase in monoamine oxidase (MAO) activity was observed in young male rats exposed to manganese daily over the early postnatal period. The rats were exposed to manganese chloride tetrahydrate in distilled water (50 µg MnCl2•4H2O daily per rat; one dose tested) via gavage for various durations (15, 30, 45 or 60 days). An increase of monoamine oxidase (MAO) activity was observed in the brain in all treated groups (Chandra and Shukla, 1978). Given that MAO serves to metabolize (inactivate) monoamine neurotransmitters (noradrenaline, dopamine, epinephrine and serotonin), this finding is consistent with the results of other studies which report altered levels of neurotransmitters such as dopamine (DA) or serotonin. Decreased DA levels were reported in the striatum and hypothalamic region of young rats and mice exposed to ≥ 10 mg Mn/kg bw per day by gavage from PND 1 to 21 or from PND 1 to 24Footnote 8 (Deskin et al., 1980; Moreno et al., 2009b). A dose-response depletion of striatal DA levels was also reported in young rats exposed to 0, 50, 250, or 500 µg Mn/day by gavage from PND 1–20) (Tran et al., 2002b). This decrease in DA was accompanied by adverse functional effects on motor development and behaviour, as measured by the righting test (reflex), homing test, and passive avoidance test (Tran et al., 2002b).
Reduced striatal DA levels were reported in male and female mice exposed to 10 and 30 mg Mn/kg bw per day as manganese chloride in saline via gavage over two periods (as juveniles from PND20 to PND34 and as adults from PNW12 to PNW20) (Moreno et al., 2009b). Decreased DA levels were also reported in the brainstem of adult (3-month-old) rats following exposure to 20 mg MnCl2/kg bw per day manganese chloride by gavage for 6.5 days (Desole et al., 1997). Long-term reduction in cocaine-evoked reflux of striatal dopamine as measured by in vivo microdialysis on PND 90 was also documented following neonatal (PND 1–21) exposure in rats (750 µg Mn/day) (McDougall et al., 2008).
At higher doses (approximately 20 mg Mn/kg bw per day and above), alterations of other neurotransmitter levels (e.g., GABA, serotonin, aspartate, Gln, Glu, taurine) or neurotransmitter enzyme (AChE) activity (Deskin et al., 1981; Lipe et al., 1999; Garcia et al., 2006), impaired DA transporter binding sites, D1-like DA receptors (Dorman et al., 2000; Liu et al., 2006 ; Reichel et al., 2006; McDougall et al., 2008; Kern et al., 2010; Kern and Smith, 2011) and aberrations in neurogenesis (Wang et al., 2012) were reported in the striatum of juvenile mice and rats.
The effects of early exposure to manganese in rats on behaviour and cognition were shown to be associated with significant alterations in D1 and D2 receptor proteins and dopamine transporter (DAT) measured in the dorsal striatum, prefrontal cortex, and nucleus accumbens (Kern et al., 2010). In this study, neonate rats were exposed to 25 and 50 mg Mn/kg bw per day (as MnCl2) via micropipette over PND 1–21. The animals were subjected to a number of tests designed to assess executive function behaviours (n = 15–20 males/treatment) (details of this testing is provided in the “behavioural endpoints” subsection that follows), and immuno-histochemistry was performed on a separate cohort of animals to assess D1, D2, and DAT levels in the brain (n = 4–7 rats/treatment). Levels of D1 receptors were significantly lower in the dorsal striatum of treated animals (both dose groups), as were DAT levels in the high-dose group. No change was measured for D2 levels in the striatum. A decrease in D1 receptors and DAT was also observed in the high-dose group of the nucleus accumbens. Although D2 receptor levels did not change measurably in the striatum or nucleus accumbens, a significant increase in the high-dose group was observed in the prefrontal cortex. These results suggest that manganese exposure during development alters dopaminergic synaptic environments in brain nuclei (Kern et al., 2010).
Neurochemical changes were also described in a subsequent study investigating whether early exposure to manganese would result in neurobehavioral and neurochemical effects lasting into adulthood (Kern and Smith, 2011). In this study, neonatal rats (PND 1–21) were exposed to MnCl2 (25 or 50 mg Mn/kg bw per day, in 25% sucrose solution) via micropipette. The rats were evaluated for brain dopamine D1- and D2-like receptors and dopamine transporter (DAT) densities in the prefrontal cortex, striatum, and nucleus accumbens on PND24 (as weanlings) and PND107 (to reflect an adult stage). Results indicated that preweaning manganese exposure led to increased D1 receptor levels in the nucleus accumbens on PND107 at the 25 mg Mn/kg bw per day exposure level; however, on PND 24, D1 receptor levels were decreased at the highest dose in this tissue and were decreased at both doses in the dorsal striatum. Significantly increased D2 receptor levels (to approximately 800% of controls) were observed in the prefrontal cortex at the highest dose on both PND24 and PND107. Levels of DAT were elevated on PND 24 in the dorsal striatum and nucleus accumbens at the highest dose levels, although no change in the transporter level persisted on PND107. It is interesting to note that the blood and brain manganese concentrations on PND 107 (after preweaning exposure on PND 1–21) were not statistically different from control concentrations (despite the observed increase on PND24) (Kern and Smith, 2011), suggesting that early exposure to manganese results in effects on the dopaminergic system that persist even after levels of manganese return to pre-exposure levels. An earlier study by a different group exposed male pup mice to manganese in drinking water (5 mg MnCl2/L) for up to 180 days. A transient increase in the content of DA, NE, HVA, and tyrosine was reported in the corpus striatum (Chandra et al., 1979a). The authors suggested that this transient elevation in striatal DA and NE was related to the hyperactivity observed following 60 and 90 days of treatment (Chandra et al., 1979a).
Decreased levels of DA in the serum, cerebellum, hypothalamus, cortex, hippocampus, mid-brain and/or striatum were reported in adult rats exposed to ≥ 22.5 mg Mn/kg bw per day (as manganese chloride or sulfate) via drinking water. Alterations of the levels of neurotransmitters or neurotransmitter metabolites (GABA, serotonin, norepinephrine (NE), DA, Glu, taurine), alterations of neurotransmitter transporters, alteration of enzymatic activities (TH) were also reported in adult rats and mice exposed to estimated doses ranging from 22.5 to 2943.3 mg Mn/kg bw per day (as manganese chloride or sulfate) via drinking water or gavage for durations varying from 7 days to 8 months (Bonilla, 1978, 1980; Chandra, 1983; Eriksson et al., 1987a; Subhash and Padmashree, 1991; Miele et al., 2000; Ranasinghe et al., 2000; Spadoni et al., 2000; Calabresi et al., 2001; Centonze et al., 2001; Montes et al., 2001; Anderson et al., 2007; 2008; 2009 ; Fordahl et al., 2010; Krishna et al., 2014). Exposure of 4 male rhesus monkeys to manganese chloride tetrahydrate (20 mg/kg bw per day, in distilled water) by gavage for 18 months resulted in an increase of tissue manganese concentrations. A decrease in the levels of DA and NE in the diencephalon, the corpus striatum, the midbrain and the pons was reported; the level of 5-hydroxytryptamine (5-HT) was decreased in the midbrain. In the medulla oblongata, DA and 5-HT levels were decreased, although the brain manganese content was similar to that for controls (Chandra et al., 1979b).
Altered GABA and other neurotransmitter levels were also reported in 30-day-old weanlings and 90-day-old adult rats exposed to 10 or 20 mg Mn/kg bw per day (as manganese chloride in water) by gavage for 30 days. At the dose level of 20 mg Mn/kg bw per day, juvenile rats exhibited an increase in GABA levels in the caudate nucleus, and increased levels of aspartate, glutamate (Glu), glutamine (Gln), taurine and GABA were reported in the cerebellum of adults; no dose-response was observed in the other brain regions examined (frontal cortex and hippocampus) (Lipe et al., 1999). Similarly, an alteration in levels of GABA and other neurotransmitters (i.e., increased serotonin and GABA levels and decreased acetylcholinesterase (AchE) levels) in the striatum, the hypothalamus or the overall brain were also reported in juvenile rats of both sexes exposed to ≥ 20 mg Mn/kg bw per day by gavage (as manganese chloride in a 5% sucrose solution; tested doses: 10, 15 and 20 µg Mn/kg bw per day) (Deskin et al., 1981) or through lactation (pups exposed PND 4–21, where dams were fed with a manganese supplemented diet at 100 ppm Mn) and then through the diet when capable (i.e., PND 11) (Garcia et al., 2006).
Decreased levels of 3,4-dihydroxyphenylacetic acid (DOPAC) in the striatum and the hypothalamus, of 5-HIAA in the hippocampus, and of noradrenaline in the pons was documented in male rats exposed to manganese chloride in drinking water for 8 months (effects observed at both concentrations tested (0.1 and 1.0 mg Mn/mL) (Bonilla, 1984b).
Changes in neurotransmitter systems were suggested to vary with the duration of exposure during manganese intoxication. Such changes were observed in studies carried out by a group of researchers who exposed male pup mice to manganese chloride through lactationFootnote 9 and then drinking water containing 3 µg Mn/mL (3 mg/L) for up to 180 days (Chandra et al., 1979a) or male rats to drinking water containing 1 mg/mL (1000 mg/L) for up to 360 days (Chandra and Shukla, 1981). Manganese exposure increased the levels of DA, NE, HVA and tyrosine in the corpus striatum (Chandra et al., 1979a; Chandra and Shukla, 1981). It should be noted, however, that this increase was transient (levels declined under the control values after 300 and/or 360 days of treatment) (Chandra and Shukla, 1981). These alterations were not correlated with the level of manganese in the corpus striatum, which gradually increased up to 120 days in mice and up to 240 days in rats, and then remained constant (Chandra et al., 1979a; Chandra and Shukla, 1981). However, the altered levels of neurotransmitters were associated with the increased activity of MAO in the striatum of rats during early periods of manganese exposure (from day 15 to day 120 of treatment). In mice, the authors proposed that the initial elevation of striatal DA and NE levels might be related to the hyperactivity observed after 60 and 90 days of exposure (Chandra et al., 1979a). Similar results (transient elevated levels of DA in the caudate-putamen) were reported in rats exposed to a higher dose of manganese (2,8 mg/L) as manganese chloride tetrahydrate in drinking water (Eriksson et al., 1987a).
It has been suggested that manganese-induced alterations of rat brain synaptosomal uptake of amines may be age-dependent. In studies by Lai et al. (1982; 1984), rats were exposed in utero and throughout life to 1 mg MnCl2•4H2O/mL manganese chloride in drinking water, corresponding to doses estimated at 33 mg Mn/kg bw per day (Lai et al., 1982Footnote 10) or 36.1 mg Mn/kg bw per day (Lai et al., 1984Footnote 11). In rats that were 70–90 days of age, the authors observed a transient decrease of DA uptake by synaptosomes isolated from the hypothalamus, the striatum and the midbrain, and a decrease of synaptosomal choline uptake in the hypothalamus. In older rats (aged 90–120 days), the synaptosomal choline uptake was increased in the striatum and the hypothalamus, though no changes were observed in DA uptake.
10.2.2 Reproductive toxicity
10.2.2.1 Adult male reproductive system
Manganese was found to alter testicular functions and to induce testicular damages after short- and long-term exposures.
In male CD-1 mice exposed for 43 days to manganese acetate (7.5, 15.0 and 30.0 mg/kg bw per day), decreased testicular sperm counts (≥ 7.5 mg /kg bw per day) and decreased sperm motility (≥ 15.0 mg/kg bw per day) were observed; however, the ability of the exposed males to impregnate unexposed females was not impaired (Ponnapakkam et al., 2003a). Increased sperm head abnormalities and percentage of abnormal sperm were also noted in mice administered potassium permanganate (65–380 mg/kg bw per day) or manganese sulfate (102.5–610 mg/kg bw per day) by gavage for 21 days (Joardar and Sharma, 1990).
Testicular damage was reported in both monkeys and rats. In adult rhesus monkeys exposed orallyFootnote 12 to manganese chloride at 25 mg MnCl2•4H2O/kg bw per day for 18 months, the authors reported congested and swollen testes accompanied by a marked increase in testis weight, interstitial oedema, and degeneration of seminiferous tubules. Leydig cells did not show any abnormality. Activities of several enzymes (succinic dehydrogenase, glucose-6-phosphate dehydrogenase and acid phosphatase) were significantly inhibited in the seminiferous tubules. The authors of this study concluded that chronic exposure to manganese did not produce severe degenerative changes in the testis earlier than metal-induced encephalopathy in primates (Murthy et al., 1980).
In Sprague-Dawley rats exposed to manganese acetate for 63 days, mild to moderate segmental degeneration of germinal epithelium within the seminiferous tubules occurred at ≥ 612 mg Mn/kg bw per day (no effect at 306 mg Mn/kg bw per day). These changes appeared to be reversible on cessation of manganese exposure (Ponnapakkam et al., 2003c).
Impaired male fertility was observed in male mice exposed to manganese chloride in drinking water (8000 mg MnCl2/L, corresponding to doses of 706 mg MnCl2/kg bw per day or 309 mg Mn/kg bw per day if the dose reported by the authors reflects MnCl2 and not elemental Mn) for 12 weeks before mating with unexposed females (no effect observed at 1000, 2000 and 4000 mg MnCl2/L) (Elbetieha et al., 2001).
10.2.2.2 Adult female reproductive system
Several studies have been conducted in rats, mice and rabbits to assess the toxicity of manganese on the female reproductive system. Impaired fertility, increased post-implantation loss and decreased fetal viability were observed in some studies at elevated doses.
Decreased numbers of implantations and viable fetuses were observed in female Swiss mice exposed to manganese chloride tetrahydrate in drinking water (8000 mg MnCl2/L) for 12 weeks before mating with unexposed males (no effect 1000, 2000 or 4000 mg MnCl2/L) (Elbetieha et al., 2001). Increased post-implantation loss was reported in pregnant rats given drinking water with concentrations of 8000 mg Mn/L (Elbetieha et al., 2001). In contrast, no reproductive effect was observed in pregnant rabbits administered similar doses of manganese chloride on GD6–GD20 (Szakmáry et al., 1995).
Manganese did not adversely affect the health of the dams, the litter size or the sex ratios of the pups when pregnant rats were administered manganese chloride in drinking water (2 or 10 mg MnCl2/mL) throughout gestation; more extensive analyses of female reproductive organs were not performed (Pappas et al., 1997). In another study, a decrease of the litter weight was reported in female rats exposed to manganese chloride through drinking water (20 mg MnCl2/mL) (Kontur and Fechter, 1985); at this dose, water intake and body weight gain by the dams were severely reduced.
No effect of manganese on maternal weight gain, implantation number, resorptions, or percentage of dead fetuses was reported in female Sprague-Dawley rats administered varying doses manganese sulfate in the diet (4, 24, 54, 154, 504 and 1004 mg Mn/kg dry diet) from weaning to mating until GD 21 (Jarvinen and Ahlström, 1975).
10.2.3 Developmental toxicity
A number of studies have investigated developmental toxicity resulting from exposure to manganese in rats, mice or rabbits using comparable exposure periods over gestation and the postnatal period. Manganese readily crosses the placental barrier and is secreted in breast milk (Saric, 1986). Neonates are particularly sensitive to manganese exposure during development, as there is evidence that they absorb and accumulate more manganese, and excrete less due to their underdeveloped capacity for biliary excretion (Deskin et al., 1980; Dorman et al., 2000; Keen et al., 1986).
Oral and/or inhalation exposure to manganese during gestation, during lactation and through the postnatal period was shown in a number of studies to alter growth and/or survival, alter the reproductive performance, and lead to neurotoxic effects. Exposure to manganese during gestation has also been linked to decreased pup body weight gain. This review focuses primarily on the developmental toxicity resulting from oral exposure to manganese. A detailed account of toxicity resulting from inhalation exposures is provided in Health Canada (2010).
10.2.3.1 Altered growth and survival
No clearly discernible effect on implantation or on maternal and fetal survival was found in two studies investigating the developmental effects of soluble forms of manganese on rats, mice, hamsters and rabbits exposed to manganese sulfate monohydrate by gavage for 5 to 13 consecutive gestation days (mice and rats GD 6–15, hamsters GD 6–10, rabbits GD 6–18; doses ranged from 0.783–1.36 (low) to 78.3–136.0 (high) mg/kg bw per day, depending on the species studied (NTIS, 1973).
In mice exposed to manganese chloride through the diet (0, 32, 160 or 800 ppm of Mn) from GD10 to PND21, reduced body weight was observed in male pups at the 160 ppm level (from PND21 to PND70) and at 800 ppm (from PND17 to PND63). In contrast, female pups showed no changes in body weight from PND2 to PND77 (Wang et al., 2012).
10.2.3.2 Altered development of the reproductive system
Manganese-induced precocious pubertal development was reported in male and female rats exposed to 10–25 mg/kg MnCl2 manganese by gavage early in postnatal development (Pine et al., 2005; Lee et al., 2006; Hiney et al., 2011).
Failure in reproductive performance as well as in neonatal growth and survival was reported in a study that exposed rats to manganeseFootnote13 in the diet (diet with basal manganese levels of 50 mg/kg bw per day plus 0, 500 or 1000 mg/kg bw per day, on a dry basis) for up to 7.5 months of treatment. As summarized in Gerber et al. (2002), histological studies revealed a reduction of spermatogenesis, epithelial alteration, atretic (degenerated) ovarian follicles, and persistence of the corpus luteum, indicative of ovarian dysfunction.
A decrease in male sexual development, as measured by decreased weights of testes, seminal vesicles and preputial organs was seen in mice given 1050 ppm of manganese in the diet as Mn3O4 who were on Fe-deficient diets (Gray and Laskey, 1980).
10.2.3.3 Teratogenicity
Only two studies investigated the teratogenicity of soluble forms of manganese administered orally and neither indicated increased abnormalities in either soft or skeletal tissues (NTIS, 1973; Grant et al., 1997). The first study was conducted in rats, mice, hamsters and rabbits exposed by gavage to manganese sulfate monohydrate for 5 to 13 consecutive gestation days (mice and rats GD 6–15, hamsters GD 6–10, rabbits GD 6–18; doses ranged from 0.783–1.36 [low] to 78.3–136.0 [high] mg/kg bw per day, depending on the species; NTIS, 1973). The second study exposed rats to manganese chloride by gavage on GD 6–17 (400 µmol/kg bw per day; Grant et al., 1997).
10.2.3.4 Developmental neurotoxicity
Exposure to manganese during gestation, during lactation and/or through the postnatal period can result in several types of neurotoxic effects, including behavioural effects that are supported by observed neurochemical and neurostructural alterations in animals, as discussed in detail in Section 9.2.2. Reported effects include altered cognitive and behavioural functions that are consistent with developmental effects reported in epidemiological studies.
Neonates are particularly sensitive to manganese exposure during postnatal development, and there is substantial evidence that neonates absorb and retain more manganese than adults, leading to greater brain manganese levels and neurotoxicity during this period (Deskin et al., 1980; Kontur and Fechter, 1985; Keen et al., 1986; Kristensson et al., 1986; Brenneman et al., 1999; Dorman et al., 2000; Weber et al., 2002; Tran et al., 2002a). This increased susceptibility is likely due to several factors, including elevated absorption of manganese from the gastrointestinal tract, an incompletely formed blood-brain barrier, the absence of biliary manganese excretion until weaning, and a greater propensity for disruption in the activity of various enzymes and impaired neurotransmitter systems (Kostial et al., 1978; Shukla et al., 1980; Kristensson et al., 1986; Brenneman et al., 1999; Dorman et al., 2000; Moreno et al., 2009b; Health Canada, 2010). This last difference between developing animals and adults was observed in studies that have compared the effects of manganese exposure in neonate and adult animals (Kostial et al., 1978; Shukla et al., 1980; Dorman et al., 2000; Moreno et al., 2009b).
The effect of manganese on motion at higher doses was observed in a three-generation study in mice: abnormal motion was reported in the gait of 2 neonates of the 3rd generation litter exposed to 25 mg Mn/kg bw per day (Ishizuka et al., 1991).
10.2.4 Other effects
The effects of dietary exposure (excluding neurotoxicity) to manganese are documented in a study conducted by the NTP (1993) in rats and mice fed manganese sulfate monohydrate in the diet for 14 days, 13 weeks, or 2 years. In the 2-year study, mice were exposed to 1500 to 15,000 ppm in diet. The doses corresponding to the higher exposure level were not adjusted for body weight and therefore varied with growth (body weight increase) of the animals. The estimated range over the course of the study was from 1171 mg/kg bw per day (week 4) to 505 mg/kg bw per day (week 104) in male rats (1249–561 mg/kg bw per day in female rats). Similarly, doses corresponding to the higher dose level in mice ranged from 2263–1916 mg/kg bw per day in males and 3449–1983 mg/kg bw per day in females. In this study, no significant effect was observed on the respiratory system, the cardiovascular system, the liver, the eyes, the muscles or the bones (pathological lesions) in either species (NTP, 1993). It should be noted that exposure in this study was through diet and not drinking water. As described in the Kinetics section of this assessment, the bioavailability of manganese exposure through drinking water (assuming a fasted state) is expected to be greater than through food.
10.2.4.1 Effects on bodyweight
Several studies conducted under a variety of conditions reported reduced body weight following oral exposure of rodents to manganese. In neonatal rats, significantly lower body weight was observed on PND21 following gavage with 250 or 750 µg Mn/day (as manganese chloride in a 10% sucrose solution) from PND1 to PND21 (Reichel et al., 2006). Reduced growth and body weight gain were also observed in neonate rats after oral exposureFootnote14 to manganese chloride at doses of 25 or 50 mg/kg bw per day on PND 1–49 and on PND 21–49 (Brenneman et al., 1999; Dorman et al., 2000). In 90-day-old rats exposed to 10 or 20 mg Mn/kg bw per day by gavage (as manganese chloride in distilled water) for 30 days, decreased body weight was observed in both groups (Lipe et al., 1999). Higher doses (≥ 120 mg Mn/kg bw per day) of manganese in diet or drinking water were also shown to induce a decrease in body weight gain in rats and mice (Komura and Sakamoto, 1991; NTP, 1993; Torrente et al., 2005b; Avila et al., 2008).
In the NTP study (1993), mean body weight was decreased in female mice exposed to ≥ 1500 ppm manganese through diet and in male rats exposed at the 15,000 ppm level. No effects on body weight were observed in male mice or in female rats (NTP, 1993).
10.2.4.2 Respiratory effects
Manganese has been shown to have effects on the respiratory tract following oral exposure (reduced lung weight) and inhalation exposure (nasal and pulmonary inflammation). A detailed assessment of the toxicological effects resulting from inhaled manganese is provided in Health Canada’s Human Health Risk Assessment for Inhaled Manganese (Health Canada, 2010).
10.2.4.3 Gastrointestinal effects
Guinea pigs administered 10 mg/kg manganese chloride by gavage for 30 days had patchy necrosis in both the stomach and small intestine with decreased cellular adenosine triphosphatase and glucose 6-phosphatase activities (Chandra and Imam, 1973). However, it is possible that the gavage treatment in this study may have partially or completely contributed to the adverse effects seen in the stomach and small intestine of these animals. Hyperplasia of the forestomach associated with ulcers and inflammation (mostly in males) was observed in mice (but not in rats) fed with 15,000 ppm manganese sulfate monohydrate in the diet for 2 years (NTP, 1993).
In male mice exposed to manganese sulfate monohydrate (up to 50,000 ppm) in the diet for 13 weeks, mild hyperplasia and hyperkeratosis of the forestomach were observed in 3/10 animals at the highest tested dose (1950 mg Mn/kg bw per day). In contrast, no gastrointestinal effects were observed in female mice exposed under similar conditions, nor in rats fed up to 25,000 ppm (NTP, 1993).
10.2.4.4 Cardiovascular effects
In two studies on weanling male Sprague-Dawley rats, dietary exposure to manganese (45–50 ppm in diet) for 15 weeks reduced the maximal vessel contraction (Kalea et al., 2005). It also led to aortic structural alterations (increase of the level of uronic acid, increased concentrations of total galactosaminoglycans and decreased concentration of hyaluronan and heparan sulfate in aortas) (Kalea et al., 2006).
10.2.4.5 Hematological effects
In rats (particularly in males), an increase of total leukocyte and neutrophils counts was observed following dietary exposure to 50,000 ppm manganese sulfate monohydrate for 14 days. Hematological effects were also reported in rats following 13 weeks of exposure (increased neutrophils counts from 1600 ppm in males and from 6250 ppmFootnote 15 in females) and mice (decreased hematocrit and hemoglobin concentrations at 50,000 ppm in males and females, respectively) (NTP, 1993).
No notable difference was observed in the hematology of rats and mice exposed to manganese though diet for 2 years; increased hematocrit, hemoglobin and red blood cell (RBC) count were the only hematological effects observed in 15,000 ppm male mice at the 15-month interim evaluation but their significance is uncertain (NTP, 1993). These results contrast with those obtained in mice fed diets containing 2 g Mn/kg for 100 days: RBC levels were decreased following exposure to manganese acetate and chloride; the white blood cell count was decreased by manganese acetate, chloride and dioxide (Komura and Sakamoto, 1991). A decrease in hematocrit was observed following exposure to manganese carbonate (Komura and Sakamoto, 1991).
10.2.4.6 Hepatic effects
Exposure of rats to 16 mg MnCl2•4H2O/kg bw per day (in a drinkable 10% sucrose solution) for 30 days induced alterations of several hepatic and serum enzyme levels (in the liver: decreased activities of succinic dehydrogenase and alcohol dehydrogenase and increased activities of monoamine oxidase (MAO), adenosine triphosphatase, arginase, alanine aminotransferase, ribonuclease and glucose-6-phosphatase; in the serum: increased activities of α-amylase and decreased activities of β-amylase) (Shukla et al., 1978). In another study, female rats exposed to higher doses of manganese in drinking water as manganese chloride tetrahydrate (1000, 10,000 or 20,000 mg MnCl2/L) from conception through the age of 60 days, exhibited liver necrosis and ultrastructural alterations (Leung et al., 1982). In the NTP study (1993), reduced relative liver weight was reported in male rats fed manganese sulfate monohydrate at ≥ 12,500 ppm for 14 days. After 13 weeks exposure, decreased liver weights were also reported in male rats at ≥ 35.8 mg Mn/kg bw per day (≥ 1,600 ppm) and in female rats at 650 mg Mn/kg bw per day (25,000 ppm). In mice, only males exhibited a relative and absolute reduction of liver weights at 2410 mg Mn/kg bw per day (50,000 ppm) (NTP, 1993).
10.2.4.7 Renal effects
Male Sprague-Dawley rats orally treated (gavage) with high levels of manganese (306–1838 mg Mn/kg bw per day as manganese acetate) for 63 days developed severe renal disease (glomerulosclerosis/nephritis and urolithiasis [kidney stones]; Ponnapakkam et al., 2003b). Female animals did not show significant differences in tissue effects relative to controls, suggesting that males are more sensitive to this manganese-induced effect. Contrary to these findings, no significant renal histopathological changes were observed in mice and rats fed elevated doses of manganese sulfate (up to 2410 and 650 mg Mn/kg bw per day, respectively) for 13 weeks (NTP 1993), nor in mice and rats fed manganese sulfate (up to 50,000 ppm in the diet) for 14 days (NTP, 1993). An increase in oxidative stress was not detected in the kidneys of rats exposed via drinking water to up to 25,000 ppm of MnCl2 (estimated as 861 mg Mn/kg bw per day) for 30 days (Avila et al., 2008).Footnote 16
A marginal increase in the average severity of nephropathy (versus control) was noted in male rats fed manganese sulfate monohydrate (15,000 ppm) for 2 years, while no significant renal histopathological changes were observed in female rats nor in mice of both sexes exposed under similar conditions (NTP, 1993).
10.2.4.8 Neuroendocrine and endocrine effects
The effect of manganese (manganese chloride) on hormonal status was investigated by a group of researchers that conducted several studies with similar designs. The reported effects include an increase of gonadotropin-releasing hormone (GnRHFootnote 17), luteinizing hormone (LH), follicle-stimulating hormone (FSH) and estradiol or testosterone levels in immature Sprague-Dawley rats exposed to MnCl2 by gavage before puberty: effects were reported in females exposed to 10 mg MnCl2/kg bw per day from PND12 to PND29 and at 25 mg MnCl2/kg bw per day in males exposed from PND15 to PND48 or from PND15 to PND55 (Pine et al., 2005; Lee et al., 2006). At these doses, continued manganese exposure also induced a moderate but significant advancement in the age of vaginal opening in females (1.5 days) and an increase of both daily sperm production and efficiency of spermatogenesis in males (Pine et al., 2005; Lee et al., 2006).
In another study, the same group of researchers investigated the effects of prepubertal manganese exposure by gavage (saline solution; 10 mg MnCl2/kg bw) of females (from PND12 to PND22 or from PND12 to PND29) on specific glial-derived puberty-related genes known to affect neuronal LH-releasing hormone (LHRH) release in the medial basal hypothalamus (MBH) and in the preoptic area / anteroventral periventricular (POA/AVPV) region. The authors noted increased expression of insulin-like growth factor-1 (IGF-1) and cyclooxygenase-2 (COX-2) genes in the MBH at PND22 and PND29, as well as of the LHRH gene in the POA/AVPV region at PND29 (Hiney et al., 2011).
The sensitivity of females to manganese during puberty was evidenced by the results of in vitro studies on sectioned basal hypothalami of adult male rats and of immature male and female rats (Pine et al., 2005; Lee et al., 2006; Prestifilippo et al., 2007). Manganese chloride stimulated LHRH release in adult males at a higher concentration (500 µM) than the one required in immature females (50 µM) and males (250 µM), respectively. Thus, the immature females more sensitive to this manganese-induced effect than immature and mature males (Pine et al., 2005; Lee et al., 2006; Prestifilippo et al., 2007).
In a 1993 NTP study, no manganese-related endocrine effects (histopathological lesions) were found in mice and rats fed manganese sulfate monohydrate (up to 25,000 ppm in rats and up to 50,000 ppm in mice) for 13 weeks. In mice fed manganese sulfate monohydrate (15,000 ppm) for 2 years, increased incidences of thyroid follicular hyperplasia and thyroid follicular dilatation were observed in both males and females; no endocrine effects were noted in rats exposed under similar conditions (NTP 1993).
10.2.4.9 Carcinogenicity
In 1996, the U.S. EPA indicated that manganese was “not classifiable as a human carcinogen” (Group D) because existing studies were considered inadequate to assess its carcinogenicity (U.S. EPA, 1996; 2014). To date, the International Agency for Research on Cancer (IARC) has not evaluated the carcinogenicity of manganese.
Clear evidence of carcinogenicity has not been established (Assem et al., 2011). In an NTP study, mice and rats were administered manganese sulfate monohydrate in their diet (0, 1500, 5000 or 15,000 ppm) for 2 years (NTP 1993). In rats of both genders (exposure up to 615/715 mg/kg bw per day for males/females), there was no evidence of carcinogenic activity. In mice of both genders (exposure up to 1800 and 2250 mg/kg bw per day for males and females, respectively), there was equivocal evidence of carcinogenicity based on a marginally increased incidence of thyroid gland follicular cell adenoma and a statistically significant increase in the incidence of follicular cell hyperplasia.
10.3 Genotoxicity
The genotoxic potential of manganese is not well understood, and results of genetic toxicity testing seem to depend upon the test system and associated protocol used. The results of in vitro studies have shown that at least some chemical forms of manganese (such as manganese sulfate) have a mutagenic/genotoxic potential. However, no overall conclusion can be made about the possible genotoxic hazard to humans from exposure to manganese compounds because results of in vivo studies in mammals are inconsistent (European Commission, 2000; Health Canada, 2010; Assem et al., 2011).
10.3.1 In vitro findings
10.3.1.1 Mutagenicity and cell transformation
In the Ames Salmonella mutagenicity assay carried out with and without metabolic activation, manganese sulfate was not mutagenic to S. typhimurium strains TA97, TA98, TA100, TA1535, or TA1537 (Mortelmans et al., 1986) but it was mutagenic to strain TA97 in another study (Pagano and Zeiger, 1992). Manganese chloride was not found to be mutagenic to S. typhimurium strains TA98, TA100 and TA1535, but it was mutagenic to strain TA1537 (Wong, 1988; de Méo et al., 1991). Both manganese sulfate and chloride were positive when tested with strain TA102 without metabolic activation (de Méo et al., 1991). The lack of mutagenicity of manganese in some of the Ames assays may be due to the lack of bioavailability of the metal ion because components of the culture media have been shown to inhibit the mutagenic activity of metal ions by chelating them or because of competition for active transport sites (NTP, 1993).
Manganese chloride tested positive without metabolic activation in the mouse lymphoma assay (80, 60, and 40 µg/mL) (Oberly et al., 1982) and in the Comet assay (single cell gel assay) performed in cultured human lymphocytes (mutagenic potency of 2.4 revertant/nmole MnCl2) (de Méo et al., 1991). It also induced cell transformations in Syrian hamster embryo cells (0.13 mM MnCl2) (Casto et al., 1979).
Manganese sulfate was slightly mutagenic and convertigenic when tested for the induction of gene conversion and reverse mutation in the strain D7 of Saccharomyces cerevisiae (Singh, 1984). In contrast it was negative for gene conversion in two other studies in yeast (Baranowska et al., 1977; Parry, 1977).
10.3.1.2 Sister chromatid exchange and chromosomal aberrations
Manganese sulfate (12,500 ppm) induced sister chromatid exchange (SCE) without metabolic activation in mouse fibroblasts (Andersen, 1983), Chinese hamster ovary (CHO) cells (Galloway et al., 1987) and human lymphocytes (Andersen, 1983) and with metabolic activation in CHO cells (NTP (1993)). Manganese sulfate also induced chromosomal aberrations without metabolic activation in CHO cells (Galloway et al., 1987; NTP 1993).
In contrast, potassium permanganate did not cause chromosomal aberrations in a primary culture of cells from Syrian hamster embryos when tested without metabolic activation (Tsuda and Kato, 1977).
10.3.2 In vivo findings
10.3.2.1 Mutagenicity
Manganese sulfate did not induce sex-linked recessive lethal mutations in Drosophila melanogaster (Valencia et al., 1985; NTP, 1993). Manganese chloride did not produce somatic mutations in D. melanogaster (Rasmuson, 1985).
No heritable translocations (in mice) or dominant lethal mutations (in rats) were detected following administration of manganese sulfate in the diet for 7 weeks in mice or by gavage once a day for 1 to 5 days in rats (Newell et al., 1974 as cited in NTP 1993).
10.3.2.2 Chromosomal aberration
Swiss albino mice orally exposed to manganese sulfate (ranging from 33 to 132 mg Mn/kg bw per day) for 3 weeks presented an increase of sperm head abnormalities and of the frequency of chromosomal aberrations and micronuclei in bone marrow cells (Joardar and Sharma, 1990). In a similar study, Swiss albino mice orally exposed to 22.6, 45.2 and 132.1 mg Mn/kg bw per day (as potassium permanganate) for three weeks exhibited an increase of sperm head abnormalities and in frequency of chromosomal aberrations in bone marrow cells (Joardar and Sharma, 1990).
No significant chromosomal damage occurred in either bone marrow or spermatogonial cells of male rats orally exposed to 0.014 mg Mn/kg bw per day (as manganese chloride) for 180 days (Dikshith and Chandra, 1978).
10.3.3 Epigenetics
Growing evidence suggests that exposure to toxicants such as metals early in life may influence gene expression later in life, possibly through epigenetics (Wright and Baccarelli, 2007). The change in gene expression without changes to DNA sequence has been suggested to ultimately alter the protein products of these genes. There is currently a knowledge gap surrounding whether elevated manganese exposure causes epigenetic modifications to DNA that may lead to altered gene expression, resulting in reported health effects. Exposure to manganese during development is reported to produce lasting changes in the expression of several dopamine system proteins, including D2, DAT, and catecholamine methyl transferase. These proteins are epigenetically regulated through CpG island methylation by the DNA methyltransferase protein DNMT (Kern et al. 2010, 2011; Shumay et al., 2010; Day et al., 2013). Manganese is a pro-oxidant at elevated exposure levels, and oxidative stress has been shown to inhibit DMNT or result in decreased DNMT protein levels (Han et al., 2011). In addition, altered DA system function has been linked to the behavioral and cognitive deficits produced by developmental manganese exposure (Kern et al., 2010, 2011; Beaudin et al., 2013, 2015; Reichel et al., 2006; McDougall et al., 2008).
11.0 Mode of action
The central nervous system is a critical target for manganese toxicity in humans, non-human primates and rodents. As discussed in sections 9 and 10 of this document, toxicological studies have shown that oral (and/or inhalation) exposure to manganese results in a variety of deleterious effects on behavior (e.g., impaired learning and memory), on motor function (e.g., altered reflex, altered activity), and on the ultrastructure of brain tissues.
Various mechanisms have been proposed to explain manganese neurotoxicity, although data suggest it involves a number of interrelated processes – such as mitochondrial dysfunction (Gavin et al., 1992; Zheng et al., 1998), free radical formation (Desole et al., 1994; 1995; Hussain et al., 1997; Taylor et al., 2006) and neurotransmitter impairment (Chandra et al., 1979a; Deskin et al., 1980; Chandra and Shukla, 1981; Lai et al., 1982; 1984; Subhash and Padmashree, 1991; Komura and Sakamoto, 1994; Ranasinghe et al., 2000; Calabresi et al., 2001; Montes et al., 2001; Tran et al., 2002a; 2002b; Fitsanakis et al., 2006; McDougall et al., 2008; Peneder et al., 2011) –that generate radicals, disrupt oxidative phosphorylation and ATP synthesis, and lead to cellular dysfunction, apoptosis/necrosis and cell death. Manganese exposure has also been reported to result in the disruption of Fe regulation, which may contribute to the mechanism of manganese neurotoxicity (Kwik-Uribe et al., 2003; Kwik-Uribe and Smith, 2006; Reaney et al., 2006; Crooks et al., 2007).
Elevated manganese levels can disrupt the regulation and interaction of neurotransmitters (DA, Glu and GABA) in the basal ganglia (Chandra et al., 1979a; Deskin et al.,1980; Chandra and Shukla, 1981; Lai et al., 1982; 1984; Subhash and Padmashree, 1991; Komura and Sakamoto, 1994; Ranasinghe et al., 2000; Calabresi et al., 2001; Montes et al., 2001; Tran et al., 2002a; 2002b; Fitsanakis et al., 2006; McDougall et al., 2008; Burton and Guilarte, 2009; Peneder et al., 2011).
DA plays a role in the regulation of cognition, behaviour, locomotor activity, and neuroendocrine secretion (Fitsanakis et al., 2006; Farina et al., 2013; Guilarte, 2013), while dopaminergic pathways are involved in the regulation of behaviours concerned with executive function (e.g., memory, learning, attention) (Kern et al., 2010). Approximately 80% of extracellular DA is recycled into presynaptic neurons through reuptake by dopamine transporters (Na+/Cl- dependent DAT and NET) that are principally located in the caudate, putamen and nucleus accumbens. DAT activity is critical for DA homeostasis in the brain as it contributes to DA cellular efflux (Roth et al., 2013) and helps regulate the duration and the dimension of dopaminergic signaling in the brain.
Neurological deficits reported in animal studies, including rodents and non-human primates, were accompanied by altered DAT and DA receptor levels, and altered response to DA antagonists. Since DAT levels increase throughout development, reaching maximal levels in adulthood (Roth et al., 2013), it has been suggested that the cognitive and neurobehavioural effects seen in children following exposure to manganese may be related to effects on the dopaminergic system during development (Neal and Guilarte, 2013).
Glutamate (Glu) is the most abundant excitatory neurotransmitter in the brain and may play a role in various CNS functions such as cognition, learning and memory, as well as in CNS development (Fitsanakis et al., 2006). Elevated levels of manganese in astrocytes appear to mediate neurotoxicity primarily through oxidative stress and mitochondrial dysfunction, but also appears to impair the Gln-Glu metabolic coupling cycle between astrocytes and neurons (i.e., the Gln/Glu-GABA cycle), which plays a critical role in normal brain function (Erikson and Aschner, 2003; Aschner et al., 2009; Sidoryk-Wegrzynowicz et al., 2009; Farina et al., 2013; Karki et al., 2013; Sidoryk-Wegrzynowicz and Aschner, 2013a; Sidoryk-Wegrzynowicz and Aschner, 2013b). The Gln/Glu-GABA cycle is crucial for optimal CNS function because it gives rise to the amino acid neurotransmitter Glu (excitatory) and GABA (inhibitory) via dynamic astrocyte-neuron interactions (Sidoryk-Wegrzynowicz and Aschner, 2013b). Disruption of this cycle leads to changes in Glu-ergic and/or GABA-ergic transmissions and Gln metabolism (Zwingmann et al., 2003; Sidoryk-Wegrzynowicz et al., 2009; Sidoryk-Wegrzynowicz and Aschner, 2013a; Sidoryk-Wegrzynowicz and Aschner, 2013b). Furthermore, disturbed astrocytic function and metabolism may prevent the astrocytes from providing the substrates used by the neurons for neurotransmitter metabolism, leading to impaired neuronal glucose oxidation, deterioration of neuronal energy metabolism and disturbance of the neurotransmission process (Zwingmann et al., 2003; Sidoryk-Wegrzynowicz and Aschner, 2013b).
Manganese exposure may increase the frequency and amplitude of spontaneous excitatory postsynaptic potentials in the striatum, resulting from hyperactivity of corticostriatal neurons (Centonze et al., 2001; Fitsanakis et al., 2006) and/or may promote excitotoxicity through over-activation of postsynaptic Glu receptors (Fitsanakis et al., 2006). Hyperactivity of the Glu receptor N-methyl-D-aspartate may lead to an increase in energy consumption due to an influx of Na+ and Ca2+ ions that must be actively pumped out of the cell. Energy-compromised cells may allow passive release of Glu, resulting in an increased extracellular Glu concentration and consequently an abnormal excitation of striatal neurons (excitotoxicity) (Erikson and Aschner, 2003; Fitsanakis and Aschner, 2005; Fitsanakis et al., 2006).
Conflicting data have been reported for GABA, with some groups of researchers seeing manganese-induced increases in striatal GABA levels whereas other groups report no effects or a decrease in GABA levels in the striatum and frontal cortex (Fitsanakis et al., 2006; Racette et al., 2012; Martinez-Finley et al., 2013; Michalke and Fernsebner, 2014). These contradictory conclusions could result from methodological differences (e.g., route and duration of exposure, age at time of exposure) and/or from the contribution and alteration of other neurotransmitter systems (Martinez-Finley et al., 2013).
12.0 Classification and assessment
Although manganese is an essential element, deficiency of manganese is very rare given that nutritional requirements are easily met through food consumption. Concern surrounding manganese-induced toxicity pertains primarily to excess exposures, particularly in infants, who lack homeostatic controls to maintain stable tissue levels of manganese. (Costa and Aschner, 2014).
Existing studies in humans and experimental animals were considered inadequate to suggest carcinogenicity as a key endpoint of concern in the risk assessment of manganese. The U.S. EPA (1999) considers that there is “inadequate information to assess human carcinogenic potential” for manganese. Manganese has not been classified by the International Agency for Research on Cancer. Consequently, a non-cancer endpoint was selected for the risk assessment of manganese in drinking water.
The central nervous system appears to be the primary target of manganese toxicity in mammals, followed by reproductive systems. Manganese has been shown to be readily taken up into the central nervous system following oral exposure, although increased levels can also occur in other tissues including the lungs, kidneys, and testes (Aschner et al., 2005; Bock et al., 2008).
Several epidemiological studies suggest an association between exposure to manganese in drinking water and neurological effects in children (Bouchard et al., 2011; Khan et al., 2011; Roels et al., 2012; Oulhote et al., 2014). These effects include intellectual impairment and poorer neurobehavioural function (memory, attention, motor function, hyperactivity) between the lowest and highest quintiles of observed exposure to manganese in drinking water, where median levels ranged from 1 to 216 µg/L (Bouchard et al., 2011; Oulhote et al., 2014).
Limitations in these studies, including potential confounding factors, inadequate exposure estimates, risk of bias, and absence of a determination of the temporality of effects, in addition to the absence of a clear point of departure needed for dose-response analysis, prevent their use in a quantitative risk assessment. However, the results of these studies can be used to qualitatively support the choice of the key endpoint used for quantitative assessment in animals, as similar endpoints that reflect executive function behaviours have been studied in rodents (e.g., behavioural hyperactivity (measured using the open arena assessment) and learning deficits (measured using the 8-arm radial maze; Kern et al., 2010). Further, it has been suggested that the cognitive and neurobehavioural effects seen in children following exposure to manganese may be related to effects on the dopaminergic system during development (Neal and Guilarte, 2013), and mechanistic data appear to suggest common elements between rodents and non-human primates with respect to involvement of the dopaminergic system in manganese-induced neurotoxicity (Neal and Guilarte, 2013). Use of a rat model for extrapolation of developmental neurotoxicity to humans is recommended as the species of choice by the OECD (2007).
A number of animal studies have identified LOAELs following oral exposure to manganese. There has been concern in the published literature over the human relevance of some of the endpoints studied, and many of the studies only examine effects following a short duration without long-term follow-up. However, three animal studies in particular stand out collectively as closely assessing and quantifying neurological effects that are consistent with those reported in the epidemiology studies, with consideration of observed effects measured over a long term (Kern et al., 2010; 2011; Beaudin et al., 2013).
The Kern and Beaudin studies were chosen as a basis for the current risk assessment because of their thoroughness in assessing neurodevelopmental endpoints (observed neurobehavioural effects are supported with corresponding neurochemical findings) in early life that are consistent with the findings reported in epidemiological studies (Bouchard et al., 2011; Khan et al., 2011; Roehls et al., 2012; Oulhote et al., 2014). These studies identified a LOAEL of 25 mg Mn/kg bw per day for various neurological endpoints in rats. In addition to demonstrating that exposure to manganese in early life can result in behavioural and sensori-motor effects, these studies provided mechanistic support by demonstrating corresponding neurostructural and neurochemical changes. Further, Kern et al. (2011) and Beaudin et al. (2013) demonstrated the ability of manganese exposure in early life to result in effects that persist into adulthood, after levels of manganese in the brain have returned to normal.
Despite their above-mentioned strengths, it should be noted that the key studies chosen do not reflect the lowest LOAELs for neurological effects following oral exposure to manganese reported in the literature, and that benchmark dose analysis was not possible given that only two doses were tested. Other studies indicated neurotoxicity resulting from oral exposure to manganese in rats, mice, and non-human primates at levels ranging from 0.106 mg/kg bw per day in non-human primates (Golub et al., 2005) to 6.5 mg/kg bw per day in young adult rats (Vezer et al., 2005, 2007); however, these studies were not considered strong candidates for development of a risk assessment on their own (Chandra and Shukla, 1978; Chandra et al., 1979a,1979b; Deskin et al., 1980; Gupta et al., 1980; Oner and Senturk, 1995, 1996; Shukakidze et al. 2002, 2003; Tran et al., 2002b; Larishvili et al., 2009; Moreno et al., 2009a, 2009b). Various study limitations include the lack of a clear account of animal dosing, lack of information concerning long-term effects, and confounding factors that hindered the interpretation of study results. Nonetheless, these studies support the choice of neurotoxicity as the key endpoint of concern for this risk assessment.
It is important to be mindful that a number of factors may influence the extent of toxicity specific to drinking water exposure in infants, including increased bioavailability of manganese when ingested in a fasted state, differing chemical form and valence states in drinking water, and the higher absorption and increased retention of manganese in infants compared to adults (Dorothy Klimis-Zacas, 1993; Costa et al., 2014; Aschner and Aschner, 2005; Keen et al., 1986).
It should also be noted that the current risk assessment considered studies that were most appropriate in the context of establishing a guideline for manganese in drinking water. Differences in bioavailability of manganese between food and water have been acknowledged in the literature and in previous risk assessments (Ruoff, 1995; IRIS, 2002; U.S. EPA, 2004; Bouchard et al., 2011), and quantitative data concerning the differences in bioavailability of manganese between exposure sources in infants are not available. Levels of manganese in children’s hair (a biomarker of manganese exposure) have been found to correlate with exposure to manganese from drinking water, though not with exposure to manganese in food (Bouchard et al., 2011). Taking the differences in bioavailability between different routes and sources of exposure into account, in addition to the limited ability of the susceptible population to maintain manganese homeostasis, caution was exercised in selecting studies with the most appropriate route and source of exposure to manganese for derivation of a health-based value for drinking water.
Given the challenges with using the existing human epidemiological studies for quantitative risk assessment, animal studies that were representative of effects reported in humans were used to quantify risk in this assessment. The Kern et al. (2010, 2011) and Beaudin et al. (2013) studies were conducted in neonatal rats to reflect developmental risk in infants (the most sensitive subpopulation). Infants (and neonates) are particularly sensitive to the effects of manganese because of their increased capacity for absorption and reduced ability for biliary excretion. Evidence also suggests that exposure to manganese in early life, during a critical period of development of the dopaminergic system, may result in lasting neurotoxic effects in adults.
Using the most appropriate LOAEL as described above, the tolerable daily intake (TDI) for manganese is calculated as follows:
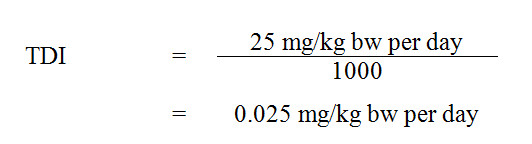
Figure 1 - Text Equivalent
The TDI for manganese is 0.025 mg/kg body weight per day. This is calculated by dividing the LOAEL of 25 mg/kg body weight per day by the uncertainty factor of 1000.
where:
- TDI is the tolerable daily intake; the dose of manganese from drinking water that is not expected to pose a risk to human health. It should be noted that significant differences exist between the bioavailability of manganese from various routes and sources of exposure (including food, air, and water);
- 25 mg/kg bw per day is the LOAEL from the Kern et al. (2010, 2011) and Beaudin et al. (2013) studies, based on neurological effects occurring from postnatal exposure to manganese in rats; and
- 1000 is the uncertainty factor, selected to account for interspecies variation (×10), intraspecies variation (×10), and the use of a LOAEL rather than a NOAEL (×10).
Using this TDI, the proposed HBV for total manganese in drinking water is calculated as follows:

Figure 2 - Text Equivalent
The HBV for manganese in drinking water is 0.1 mg/L (rounded). This is calculated by multiplying the TDI for manganese of 0.025 mg/kg body weight per day by the average infant body weight of 7 kg, then by 0.5, the allocation factor denoting the contribution of drinking water to the tolerable daily intake, and dividing the resulting product by 0.75 L per day, the daily average volume of drinking water ingested by a bottle-fed infant (0 – 6 months of age).
where:
- 0.025 mg/kg bw per day is the TDI derived above;
- 7 kg is the average body weight of an infant (0–6 months) (Health Canada, 1999);
- 0.5 is the allocation factor estimated for drinking water. Given that formula represents the total diet in non-breast fed infants for the first few months of life, potentially increased levels of manganese in both the water used to prepare formula as well as powdered formula itself are considered the main sources of exposure. Due to the high variability of manganese in drinking water and formula, the source allocation from drinking water is assumed to be half of the total potential exposure, with the balance from the formula itself. Contributions from other sources are not expected to be significant for this age group; and
- 0.75 L per day is the estimated daily volume of tap water consumed by a bottle-fed infant in the 0–6 months age group (Health Canada, 1999).
12.1 International considerations
Other agencies have established limits for manganese in drinking water. Generally, two different values are established: one for aesthetic or operational limits, and one based on human health.
The Minnesota Department of Health developed a tiered guidance document based on the same endpoint and key study (Kern et al., 2010) used by Health Canada to derive its proposed HBV. A level of 0.1 mg/L was established for infants less than 1 year old, and 0.3 mg/L for children > 1 year old and adults (MDH, 2012).
The U.S. EPA established a secondary maximum contaminant level for manganese in drinking water of 0.05 mg/L, based on aesthetic considerations. In addition, a non-regulatory health advisory of 0.3 mg/L was published, which was based on absence of effects in dietary intake studies (U.S. EPA, 2004).
The World Health Organization established a health-based value of 0.4 mg/L, based on an absence of effect in a composite of dietary studies (WHO, 2011). The WHO determined that it was not considered necessary to establish a formal guideline given that this health-based value is well above concentrations normally found in drinking water.
The Australian Drinking Water Guidelines report a health-based guideline of 0.5 mg/L, which was derived from an amount of manganese exposure that was considered safe from all sources by the WHO, and an aesthetic guideline of 0.1 mg/L for manganese in drinking water based on plant experience with respect to consumer acceptance (NHMRC, 2011).
13.0 Rationale
Manganese occurs naturally in the environment under several oxidation states. It is widely distributed in air, water and soil, and may be present in water from natural sources, such as rock and soil weathering, or as a result of human activities. Manganese is generally more prevalent in ground waters than in surface waters.
Manganese is an essential element for humans. Deficiency is considered unlikely in Canada, as adequate amounts are obtained from food. Existing studies are inadequate to determine whether manganese could be carcinogenic, and it has not been classified with respect to carcinogenicity by IARC or by the U.S. EPA. Manganese is more readily bioavailable from drinking water than from food. Levels of manganese in children’s hair correlate with exposure to manganese from drinking water, but not with exposure to manganese in food.
The central nervous system appears to be the primary target of manganese toxicity in both animals and humans. Several epidemiological studies suggest an association between exposure to manganese in drinking water and neurological effects in children, such as intellectual impairment and poorer neurobehavioural function. Although the limitations of these studies prevent their use to establish a proposed MAC, they can be used to qualitatively support the choice of the key end-point in animal studies. The reported neurological effects observed in children are consistent with the neurological effects reported in the key animal studies used as a basis for the calculation of the health-based value.
Infants are the most sensitive subpopulation to the health effects of manganese, having higher absorption and retention of manganese than adults. Although established for the most sensitive subpopulation, the HBV is also protective for chronic exposure in children and adults. In addition, exposure to manganese in drinking water at levels at or below the proposed HBV is not expected to result in other types of toxicity that have been associated with exposure to manganese. A health-based value of 0.1 mg/L has been calculated for manganese in drinking water.
A MAC of 0.1 mg/L (100 µg/L) and an AO of 0.02 mg/L (20 µg/L) are proposed for total manganese in drinking water. The proposed MAC is established at a level to be protective of neurological effects in infants, the most sensitive population, and the proposed AO will reduce consumer complaints regarding discoloured water and staining of laundry. The proposed MAC and AO for manganese can be measured by available analytical methods and are achievable by municipal and residential treatment technologies. As part of its ongoing guideline review process, Health Canada will continue to monitor new research and recommend any change to the guideline that is deemed necessary.
14.0 References
Aisen, P., Aasa, R. and Redfield, A.G. (1969). The chromium, manganese, and cobalt complexes of transferrin. J. Biol. Chem., 244(17): 4628–4633. [As cited in Health Canada, 2010]
Alberta Environment (2014). Personal communication with H. Li, J. Gawor, D. Reid and J. Benjamin.
Alessio, L., Apostoli, P., Ferioli, A. and Lombardi, S. (1989). Interference of manganese on neuroendocrinal system in exposed workers. Biol. Trace Elem. Res., 21: 249–253. [As cited in Health Canada, 2010]
Ali, M.M., Murthy, R.C., Mandal, S.K. and Chandra, S.V. (1985). Effect of low protein diet on manganese neurotoxicity: III. Brain neurotransmitter levels. Neurobehav. Toxicol.Teratol., 7: 427–431. [As cited in Health Canada, 2010]
Ali, M.M., Murthy, R.C., Saxena DK, Srivastava, R.S. and Chandra, S.V. (1983). Effect of low protein diet on manganese neurotoxicity: I. Developmental and biochemical changes. Neurobehav. Toxicol.Teratol., 5(3): 377–383. [As cited in Health Canada, 2010]
Allen, J., Kligerman, A., Campbell, J., Westbrook Collins, B., Erexson, G., Kari, F. and Zeiger, E. (1990). Cytogenetic analyses of mice exposed to dichloromethane. Environ. Mol. Mutagen., 15: 221–228.
Andersen, O. (1983). Effects of coal combustion products and metal compounds on sister chromatid exchange (SCE) in a macrophage-like cell line. Environ. Health Perspect., 47: 239–253. [As cited in NTP, 1993.]
Andersen, M.E., Gearhart, J.M. and Clewell, H.J. 3rd (1999). Pharmacokinetic data needs to support risk assessments for inhaled and ingested manganese. Neurotoxicology, 20(2): 161–171. [As cited in Health Canada, 2010]
Anderson, J.G., Cooney, P.T. and Erikson, K.M. (2007). Brain manganese accumulation is inversely related to gamma-amino butyric acid uptake in male and female rats. Toxicol. Sci., 95(1): 188–95.
Anderson, J.G., Fordahl, S.C., Cooney, P.T., Weaver, T.L., Colyer, C.L. and Erikson, K.M. (2008). Manganese exposure alters extracellular GABA, GABA receptor and transporter protein and mRNA levels in the developing rat brain. Neurotoxicology, 29(6): 1044–1053.
Anderson, J.G., Fordahl, S.C., Cooney, P.T., Weaver, T.L., Colyer, C.L. and Erikson, K.M. (2009). Extracellular norepinephrine, norepinephrine receptor and transporter protein and mRNA levels are differentially altered in the developing rat brain due to dietary iron deficiency and manganese exposure. Brain Res., 1281: 1–14.
Angara, G., Cummings, L., Knocke, W.R., and Budd, G.C. (2004). Intermediate and long-term manganese control strategies during the upgrade of the Little Falls Water Treatment Plants. Proceedings of the American Water Works Association Water Quality Technology Conference.
APHA, American Water Works Association and Water Environment Federation (1992). Standard methods for the examination of water and wastewater, 18th edition. American Public Health Association, Washington, DC.
APHA, American Water Works Association and Water Environment Federation (1995). Standard methods for the examination of water and wastewater 19th edition.. American Public Health Association, Washington, DC.
APHA, American Water Works Association and Water Environment Federation (1998). Standard methods for the examination of water and wastewater 20th edition American Public Health Association, Washington, DC.
APHA, American Water Works Association and Water Environment Federation (2005). Standard methods for the examination of water and wastewater, 21st edition. American Public Health Association, Washington, DC.APHA,
APHA, American Water Works Association and Water Environment Federation (2012). Standard methods for the examination of water and wastewater 22nd edition.. American Public Health Association, Washington, DC.
Archibald, F.S. and Tyree, C. (1987). Manganese poisoning and the attack of trivalent manganese upon catecholamines. Arch. Biochem. Biophys., 256(2): 638–650. [As cited in Health Canada, 2010]
Arnaud, J. and Favier, A. (1995). Copper, iron, manganese and zinc contents in human colostrum and transitory milk of French women. Sci. Total Environ., 159(1): 9–15. [As cited in Health Canada, 2010]
Arnich, N., Cunat, L., Lanhers, M.C. and Burnel, D. (2004). Comparative in situ study of the intestinal absorption of aluminum, manganese, nickel, and lead in rats. Biol. Trace Elem. Res., 99:(1–3): 157–171. [As cited in Health Canada, 2010]
Aschner, J.L. and Aschner, M. (2005). Nutritional aspects of manganese homeostasis. Mol. Aspects Med. 26(4–5): 353–362. Review.
Aschner, M., Vrana, K.E. and Zheng, W. (1999). Manganese uptake and distribution in the central nervous system (CNS). Neurotoxicology, 20(2–3): 173–180. [As cited in Health Canada, 2010]
Aschner, M., Erikson, K.M. and Dorman, D.C. (2005). Manganese dosimetry: Species differences and implications for neurotoxicity. Crit. Rev. Toxicol., 35(1): 1–32.
Aschner, M., Guilarte, T.R., Schneider, J.S. and Zheng, W. (2007). Manganese: recent advances in understanding its transport and neurotoxicity. Toxicol. Appl. Pharmacol., 221(2): 131–147.
Aschner, M., Erikson, K.M., Herrero Hernandez, E., Hernandez, E.H. and Tjalkens, R. (2009). Manganese and its role in Parkinson's disease: from transport to neuropathology. Neuromolecular Med., 11(4): 252–266.
Assem, F.L., Holmes, P. and Levy, L.S. (2011). The mutagenicity and carcinogenicity of inorganic manganese compounds: a synthesis of the evidence. J. Toxicol. Env. Heal. B, 14(8): 537–570.
ATSDR (2012). Toxicological profile for manganese. U.S. Department of Health and Human Services. Public Health Service. ATSDR, Atlanta, Georgia.
Au, C., Benedetto, A. and Aschner, M. (2008). Manganese transport in eukaryotes: the role of DMT1. Neurotoxicology, 29(4): 569–576.
Avila, D.S., Gubert, P., Fachinetto, R., Wagner, C., Aschner, M., Rocha, J.B., et al. (2008). Involvement of striatal lipid peroxidation and inhibition of calcium influx into brain slices in neurobehavioral alterations in a rat model of short–term oral exposure to manganese. Neurotoxicology, 29(6): 1062–1068.
AWWA (1984). Removing iron and manganese from groundwater. Confluence, 76(11): 67–69.
AWWA (1993). Committee Report: Research needs for inorganic contaminants. J. Am. Water Works Assoc., 5: 106 –113.
AWWA (2005). Microfiltration and ultrafiltration membranes for drinking water. M53 Manual of Water Supply Practices. American Water Works Association, Denver, Colorado.
Baldwin, M., Mergler, D., Larribe, F., Belanger, S., Tardif, R., Bilodeau, L. and Hudnell, K. (1999). Bioindicator and exposure data for a population based study of manganese. Neurotoxicology, 20(2–3): 343–353.
Baranowska, H., Ejchart, A. and Putrament, A. (1977). Manganese mutagenesis in yeast. V. On mutation and conversion induction in nuclear DNA. Mutat. Res., 42: 343–348. [As cited in NTP, 1993.]
Barbeau, B., Carriere, A., and Bouchard, M. (2011). Spatial and temporal variations in manganese concentrations in drinking water. J. Environ. Sci. Health A Tox. Hazard. Subst. Environ. Eng.,. 46(6): 608–616.
Barbeau, B., Gauthier, V., Julienne, K., Carrière, A. (2005). Dead-end flushing of a distribution system : Short and long-term effects on water quality. J. Water Supply Res. T., 54(6), 371–383.
Barceloux, D.G. (1999). Manganese. J. Toxicol. Clin. Toxicol., 37(2): 293–307.
Beaudin, S.A., Nisam, S. and Smith, D.R. (2013). Early life versus lifelong oral manganese exposure differently impairs skilled forelimb performance in adult rats. Neurotoxicol. Teratol., 38: 36–45.
Benskin, P. and Linder, K. (2004). Hypoliminion withdrawal renews reservoir. Opflow, 5: 12–15.
Berghem, L., Hansson, M., Lundborg, M. and Camner, P. (1987). Fibronectin concentrations in lung lavage fluid after inhalation exposure to low levels of metals. Environ. Res., 43(1): 179–185. [As cited in Health Canada, 2010]
Bertinchamps, A.J., Miller, S.T. and Cotzias, G.C. (1965). Interdependence of routes excreting manganese. Am. J. Physiol., 211: 217–224.
Betharia, S. and Maher, T.J. (2012). Neurobehavioral effects of lead and manganese individually and in combination in developmentally exposed rats. Neurotoxicology, 33(5): 1117–27.
Bhang, S., Cho, S., Kim, J., Hong, Y., Shin, M., Yoo, H.J., Cho, I.H., Kim, Y. and Kim, B. (2013). Relationship between blood manganese levels and children's attention, cognition, behavior, and academic performance: A nationwide cross-sectional study. Environ. Res., 126(0): 9–16.
Bierlein, K.A., Knocke, W.R., Tobiason, J.E., Subramaniam, Pham, M., and Little, J.C. (2015). Modeling manganese removal in a pilot-scale postfiltration contractor. J. Am. Water Works Assoc., 107(2): 109–119.
Bikashvili, T.Z., Shukakidze, A.A. and Kiknadze, G.I. (2001). Changes in the ultrastructure of the rat cerebral cortex after oral doses of manganese chloride. Neurosci. Behav. Physiol., 31(4): 385–389.
Blecharz-Klin, K., Piechal, A., Joniec-Maciejak, I., Pyrzanowska, J. and Widy-Tyszkiewicz, E. (2012). Effect of intranasal manganese administration on neurotransmission and spatial learning in rats. Toxicol. Appl. Pharmacol., 265(1): 1–9.
Bock, N.A., Paiva, F.F., Nascimento, G.C., Newman, J.D. and Silva, A.C. (2008). Cerebrospinal fluid to brain transport of manganese in a non-human primate revealed by MRI. Brain Res., 1198: 160–170.
Bonilla, E. (1978). Increased GABA content in caudate nucleus of rats after chronic manganese chloride administration. J. Neurochem., 31(2): 551–552.
Bonilla, E. (1980). L-tyrosine hydroxylase activity in the rat brain after chronic oral administration of manganese chloride. Neurobehav. Toxicol., 2: 37–41. [As cited in Newland, 1999.]
Bonilla, E. (1984a). Chronic manganese intake induces changes in the motor activity of rats. Exp. Neurol., 84(3): 696–700.
Bonilla, E. (1984b). Effects of chronic manganese intake on the levels of biogenic amines in rat brain regions. Neurobeh. Toxicol. Teratol., 6: 341–344.
Bornhorst, J., Ebert, F., Hartwig, A., Michalke, B. and Schwerdtle, T. (2010). Manganese inhibits poly(ADP-ribosyl)ation in human cells: a possible mechanism behind manganese-induced toxicity? J. Environ. Monit., 12(11): 2062–2069.
Bornhorst, J., Meyer, S., Weber, T., Böker, C., Marschall, T., Mangerich, A., et al. (2013). Molecular mechanisms of Mn induced neurotoxicity: RONS generation, genotoxicity, and DNA-damage response. Mol. Nutr. Food Res., 57(7): 1255–1269.
Bouchard, M.F., Sauve, S., Barbeau, B., Legrand, M., Brodeur, M.E., Bouffard, T., Limoges, E., Bellinger, D.C. and Mergler, D. (2011). Intellectual impairment in school-age children exposed to manganese from drinking water. Environ. Health Perspect., 119(1): 138–143.
Bowler, R.M., Gysens, S., Diamond, E., Nakagawa, S., Drezgic, M. and Roels, H.A. (2006). Manganese exposure: Neuropsychological and neurological symptoms and effects in welders. Neurotoxicology, 27(3): 315–326.
Bowman, A.B., Kwakye, G.F., Hernandez, E.H. and Aschner, M. (2011). Role of manganese in neurodegenerative diseases. J. Trace Elem. Med. Biol., 25(4): 191–203.
Brandhuber, P, Clark, S., Knocke, W., and Tobiason, J. (2013). Guidance for the treatment of manganese. Water Research Foundation, Denver, Colorado.
Brandhuber, P., Craig, S., Friedman, M.J., Hill, A., Booth, S., Hanson, A. (2015). Legacy of manganese accumulation in water systems. Water Research Foundation, Denver, Colorado.
Brenneman, K.A., Cattley, R.C., Ali, S.F. and Dorman, D.C. (1999). Manganese-induced developmental neurotoxicity in the CD rat: Is oxidative damage a mechanism of action? Neurotoxicology, 20(2–3): 477–488. [As cited in Health Canada, 2010]
British Columbia Ministry of Health (2014). Personal communication from D. Fishwick.
Brna, P., Gordon, K., Dooley, J.M. and Price, V. (2011). Manganese toxicity in a child with iron deficiency and polycythemia. J. Child Neurol., 26(7): 891–894.
Brodeur, M.E. and Barbeau, B. (2015). Performance of point-of-use and point-of-entry technologies for the removal of manganese in drinking water: Summary of the EDU-MANGO Epidemiological Study. Report prepared for Health Canada.
Bruins, J. H., Petrusevski, B., Slokar, Y.M., Huysman, K., Koen, J., Kruithof, J.C., and Kennedy, M.D. (2015). Biological and physico-chemical formation of Birnessite during the ripening of manganese removal filters. Water Res., 69: 154–161.
Burger, M.S, Krentz, C.A., Mercer, S.S., and Gagnon, G.A. (2008b). Manganese removal and occurrence of manganese-oxidizing bacteria in full-scale biofilters. J. Water Supply Res. T., 57(5): 351–359.
Burger, M.S., Mercer, S.S., Shupe, G.D., and Gagnon, G.A. (2008a). Manganese removal during bench-scale biofiltration. Water Res., 42: 4733–4742.
Burton, N.C. and Guilarte, T.R. (2009). Manganese neurotoxicity: Lessons learned from longitudinal studies in nonhuman primates. Environ. Health Perspect., 117(3): 325–332.
Burton, N.C., Schneider, J.S., Syversen, T. and Guilarte, T.R. (2009). Effects of chronic manganese exposure on glutamatergic and GABAergic neurotransmitter markers in the nonhuman primate brain. Toxicol. Sci., 111(1): 131–139.
Cahill, D.F., Bercegeay, M.S., Haggerty, R.C., Gerding, J.E. and Gray, L.E. (1980). Age-related retention and distribution of ingested Mn3O4 in the rat. Toxicol. Appl. Pharmacol., 53(1): 83–91. [As cited in Health Canada, 2010]
Calabresi, P., Ammassari-Teule, M., Gubellini, P., Sancesario, G., Morello, M., Centonze, D., et al. (2001). A synaptic mechanism underlying the behavioral abnormalities induced by manganese intoxication. Neurobiol. Dis., 9: 419–432.
Camara, E., Montreuil, K.R., Knowles, A.D. and Gagnon, G.A. (2013). Role of the water main in lead service line replacement: A case study. . J. Am. Water Works Assoc., E423–E431.
Camner, P., Curstedt, T. and Jarstrand, C. (1985). Rabbit lung after inhalation of manganese chloride: A comparison with the effects of chlorides of nickel, cadmium, cobalt, and copper. Environ. Res, 38: 301–309. [As cited in Health Canada, 2010]
Carlson, K.H., Knocke, W.R., and Gertig, K.R. (1997). Optimizing treatment through Fe and Mn fractionations. J. Am. Water Works Assoc., 89(4): 162–171.
Carrière, A., Gauthier, V., Desjardins, R., Barbeau, B. (2005). Evaluation of loose deposits in distribution systems through unidirectional flushing. J. Am. Water Works Assoc., 97(9): 82–92.
Carrière, A., Brouillon, M., Sauvé, S., Bouchard, M., and Barbeau, B. (2011). Performance of point-of-use devices to remove manganese from drinking water. J. Environ. Sci. Health A Tox. Hazard Subst. Environ. Eng., 46(6): 601–607.
Casale, R.J., LeChevallier, M.W. and Pontius, F.W. (2002). Manganese control and related issues. American Water Works Research Foundation and American Water Works Association. Denver, Colorado.
Casey, C.E., Hambidge, K.M. and Neville, M.C. (1985). Studies in human lactation: Zinc, copper, manganese and chromium in human milk in the first month of lactation. Am. J. Clin. Nutr., 41(6): 1193–1200.
Casto, B.C., Meyers, J. and DiPaolo, J.A. (1979). Enhancement of viral transformation for evaluation of the carcinogenic or mutagenic potential of inorganic metal salts. Cancer Res., 39: 193–198.
Centonze, D., Gubellini, P., Bernardi, G. and Calabresi, P. (2001). Impaired excitatory transmission in the striatum of rats chronically intoxicated with manganese. Exp. Neurol., 172(2): 469–476.
Cerrato, J.M., Reyes, L.P., Alvarado, C.N., Dietrich, A.M. (2006). Effect of PVC and iron materials on Mn(II) deposition in drinking water distribution systems, Water Res., 40, 2720–2726.
Chan, A.W., Minski, M.J., Lim, L. and Lai, J.C. (1992). Changes in brain regional manganese and magnesium levels during postnatal development: modulations by chronic manganese administration. Abstract. Metab. Brain Dis., 7(1): 21–33.
Chandra, S.V. (1983). Psychiatric illness due to manganese poisoning. Acta Psychiat. Scand., 67(Suppl 303): 49–54.
Chandra, S.V. and Imam, Z. (1973). Manganese induced histochemical and histological alterations in gastrointestinal mucosa of guinea pigs. Abstract. Acta pharmacol. Toxicol., 33: 449–458.
Chandra, S.V. and Shukla, G.S. (1976). Role of iron deficiency in inducing susceptibility to manganese toxicity. Arch. Toxicol., 35(4): 319–323. [As cited in Health Canada, 2010]
Chandra, S.V. and Shukla, G.S. (1978). Manganese encephalopathy in growing rats. Environ. Res., 15: 28–37.
Chandra, S.V. and Shukla, G.S. (1981). Concentrations of striatal catecholamines in rats given manganese chloride through drinking water. J. Neurochem., 36: 683–687.
Chandra, S.V., Shukla, G.S. and Saxena, D.K. (1979a). Manganese-induced behavioral dysfunction and its neurochemical mechanism in growing mice. J. Neurochem., 33: 1217–1221.
Chandra, S.V., Srivastava, R.S. and Shukla, G.S. (1979b). Regional distribution of metals and biogenic amines in the brain of monkeys exposed to manganese. Toxicol. Lett., 4(3): 189–192.
Charlton, N., Tikos, E., Gaffney, L. and Kohl, P. (2002). Breakpoint breakdown: optimization of manganese removal to very low levels with oxide-coated filter media. Proceedings of the American Water Works Association Water Quality Technology Conference.
Chen, P., Chakraborty, S., Mukhopadhyay, S., Lee, E., Paoliello, M.M., Bowman, A.B., Aschner, M. (2015). Manganese homeostasis in the nervous system. J. Neurochem., 134(4): 601–610.
Chen, J.Y., Tsao, G.C., Zhao, Q. and Zheng, H.S. (2001). Differential cytotoxicity of Mn(II) and Mn(III): Special reference to mitochondrial [Fe-S] containing enzymes. Toxicol. Appl. Pharmacol., 175(2): 160–168. [As cited in Health Canada, 2010]
Chen, L., Ding, G., Gao, Y., Wang, P., Shi, R., Huang, H. and Tian, Y. (2014). Manganese concentrations in maternal-infant blood and birth weight. Environ. Sci. Pollut. Res. Int., 21(9): 6170–6175.
Clark, N.A., Teschke, K., Rideout, K. and Copes, R. (2007). Trace element levels in adults from the west coast of Canada and associations with age, gender, diet, activities, and levels of other trace elements. Chemosphere, 70(1): 155–164.
Cohen, J.M., Kamphake, L.J., Harris, E.K. and Woodward, R.L. (1960). Taste threshold concentrations of metals in drinking water. J. Am. Water Works Assoc., 52: 660.
Committee on Nutrition, American Academy of Pediatrics (2003). In: Kleinman, R.E. (ed.), Pediatric Nutrition Handbook, 5th edition.
Corella-Vargas, R. (1984). [High levels of manganese in the diet of rats (Rattus norvegicus albinicus). I. Effect on reproduction] (Spanish). Arch. Latinoam. Nutr., 34: 457–65. [As cited in Gerber et al., 2002.]
Corkal, D.R., Braul, L., Poon, D., Hicks, W. and Germin, G. (2005). Piloting small rural systems for Fe, Mn and TDS removal. Proceedings of the 57th Annual Conference of the Western Canada Water and Wastewater Association, Saskatoon, Saskatchewan.
Costa, L., Aschner, M., eds. (2014). Manganese in health and disease. RSC Publishing.
Cotzias, G.C., Horiuchi, K., Fuenzalida, S. and Mena, I. (1968). Chronic manganese poisoning. Clearance of tissue manganese concentrations with persistence of the neurological picture. Neurology, 18(4): 376–382. [As cited in Health Canada, 2010]
Cotzias, G.C., Miller, S.T., Papavasiliou, P.S. and Tang, L.C. (1976). Interactions between manganese and brain dopamine. Med. Clin. North Am., 60(4): 729–738. [As cited in Health Canada, 2010]
Covy, J.P. and Giasson, B.I. (2011). Alpha-Synuclein, leucine-rich repeat kinase-2, and manganese in the pathogenesis of Parkinson disease. Neurotoxicology, 32(5): 622–9.
CRC Handbook of Chemistry and Physics (1983). 64th edition. CRC Press.
Criswell, S.R., Perlmutter, J.S., Videen, T.O., Moerlein, S.M., Flores, H.P., Birke, A.M. and Racette, B.A. (2011). Reduced uptake of [18F]FDOPA PET in asymptomatic welders with occupational manganese exposure. Neurology, 76(15): 1296–1301.
Critchfield, J.W. and Keen, C.L. (1992). Manganese+2 exhibits dynamic binding to multiple ligands in human plasma. Metabolism 41(10): 1087–1092. [As cited in Health Canada, 2010]
Crooks, D.R., Ghosh, M.C., Braun-Sommargren, M., Rouault, T.A. and Smith, D.R. (2007). Manganese targets m-aconitase and activates iron regulatory protein 2 in AF5 GABAergic cells. J. Neurosci. Res., 85(8): 1797–809.
Crossgrove, J.S. and Yokel, R.A. (2004). Manganese distribution across the blood-brain barrier. III. The divalent metal transporter-1 is not the major mechanism mediating brain manganese uptake. Neurotoxicology, 25(3): 451–460. [As cited in Health Canada, 2010]
Crossgrove, J.S. and Yokel, R.A. (2005). Manganese distribution across the blood-brain barrier. IV. Evidence for brain influx through store-operated calcium channels. Neurotoxicology, 26(3): 297–307. [As cited in Health Canada, 2010]
Crossgrove, J.S., Allen, D.D., Bukaveckas, B.L., Rhineheimer, S.S. and Yokel, R.A. (2003). Manganese distribution across the blood-brain barrier I. Evidence for carrier-mediated influx of manganese citrate as well as manganese and manganese transferrin. Neurotoxicology, 24(1): 3–13. [As cited in Michalke et al., 2007.]
Davidsson, L., Cederblad, A., Hagebo, E., Lonnerdal, B. and Sandstrom, B. (1988). Intrinsic and extrinsic labeling for studies of manganese absorption in humans. J. Nutr., 118(12): 1517–1521.
Davidsson, L., Cederblad, A., Lönnerdal, B. and Sandström, B. (1989a). Manganese retention in man: A method for estimating manganese absorption in man. Am. J. Clin. Nutr., 49(1): 170–179.
Davidsson, L., Lönnerdal, B., Sandström, B., Kunz, C. and Keen, C.L. (1989b). Identification of transferrin as the major plasma carrier protein for manganese introduced orally or intravenously of after in vitro addition in the rat. J. Nutr., 119(10): 1461–1464.
Davis, C.D., Malecki, E.A. and Greger, J.L. (1992a). Interactions among dietary manganese, heme iron and non-heme iron in women. Am. J. Clin. Nutr., 56: 926–932. [As cited in Health Canada, 2010]
Davis, C.D., Wolf, T.L. and Greger, J.L. (1992b). Varying levels of manganese and iron affect absorption and gut endogenous losses of manganese by rats. J. Nutr., 122: 1300–1308.
Davis, C.D., Zech, L. and Greger, J.L. (1993). Manganese metabolism in rats: An improved methodology for assessing gut endogenous losses. Proceedings of the Society for Experimental Biology and Medicine, 202(1): 103–108. [As cited in Health Canada, 2010]
Day, J.J., Childs, D., Guzman-Karlsson, M.C., Kibe, M., Moulden, J., Song, E., Tahir, A. and Sweatt, J.D. (2013). DNA methylation regulates associative reward learning. Nat. Neurosci., 16(10): 1445–1452.
Del Toral, M.A., Porter, A., Schock, M.R. (2013). Detection and evaluation of elevated lead release from service lines: a field study. Environ. Sci. Tech., 47(16): 9300–9307.
De Méo, M., Laget, M., Castegnaro, M. and Duménil, G. (1991). Genotoxic activity of potassium permanganate in acidic solutions. Mutat. Res., 260(3): 295–306. [As cited in ATSDR 2008.]
Deskin, R., Bursian, S.J. and Edens, F.W. (1980). Neurochemical alterations induced by manganese chloride in neonatal rats. Neurotoxicology, 2: 65–73.
Deskin, R., Bursian, S.J. and Edens, F.W. (1981). The effect of chronic manganese administration on some neurochemical and physiological variables in neonatal rats. Gen. Pharmacol., 12: 279–280.
Desole, M.S., Miele, M., Esposito, G., Migheli, R., Fresu, L., De Natale, G., et al. (1994). Dopaminergic system activity and cellular defense mechanisms in the striatum and striatal synaptosomes of the rat subchronically exposed to manganese. Abstract. Arch. Toxicol., 68(9): 566–570.
Desole, M.S., Esposito, G., Migheli, R., Fresu, L., Sircana, S., Miele, M., et al. (1995). Allopurinol protects against manganese-induced oxidative stress in the striatum and in the brainstem of the rat. Neurosci. Lett. 192(2): 73–76.
Desole, M.S., Esposito, G., Migheli, R., Sircana, S., Delogu, M.R., Fresu, L., et al. (1997). Glutathione deficiency potentiates manganese toxicity in rat striatum and brainstem and in PC12 cells. Pharmacol. Res, 36(4): 285–92.
Dietrich, A.M. and Burlingame, G.A. (2015). Critical review and rethinking of USEPA Secondary Standards for maintaining organoleptic quality of drinking water. Environ. Sci. Technol., 49: 708–720.
Dikshith , T.S. and Chandra, S.V. (1978). Cytological studies in albino rats after oral administration of manganese chloride. Bull. Environ. Contam. Toxicol., 19: 741–746. [As cited in Health Canada, 2010]
Dobson, A.W., Weber, S., Dorman, D.C., Lash, L.K., Erikson, K.M. and Aschner, M. (2003). Oxidative stress is induced in the rat brain following repeated inhalation exposure to manganese sulfate. Biol. Trace Elem. Res., 93(1–3): 113–126.
Dong, D., Derry, L.A., Lion, L.W. (2003). Pb scavenging from a freshwater lake by Mn oxides in heterogeneous surface coating materials. Water Res., 37: 1662–1666.
Dorman, D.C., Struve, M.F., Vitarella, D., Byerly, F.L., Goetz, J. and Miller, R. (2000). Neurotoxicity of manganese chloride in neonatal and adult CD rats following subchronic (21-day) high-dose oral exposure. J. Appl. Toxicol., 20(3): 179–187.
Dorman, D.C., Struve, M.F., Clewell, H.J., 3rd and Andersen, M.E. (2006). Application of pharmacokinetic data to the risk assessment of inhaled manganese. Neurotoxicology, 27(5): 752–764.
Dörner, K., Dziadzka, S., Höhn, A., Sievers, E., Oldigs, H.D., Schulz-Lell, G., et al. (1989). Longitudinal manganese and copper balances in young infants and preterm infants fed on breast-milk and adapted cow's milk formulas. British Journal of Nutrition, 61: 559–572. [As cited in Health Canada, 2010]
Dupont, C.L. and Tanaka, Y. (1985). Blood manganese levels in children with convulsive disorder. Biochem. Med., 33(2): 246–255.
Eastman, R. R., Jursa, T. P., Benedetti, C., Lucchini, R. G., & Smith, D. R. (2013). Hair as a biomarker of environmental manganese exposure. Environmental Science & Technology, 47(3), 1629–1637.
Elbetieha, A., Bataineh, H., Darmani, H. and Al-Hamood, M.H. (2001). Effects of long-term exposure to manganese chloride on fertility of male and female mice. Toxicol. Lett., 119(3): 193–201.
Environment Canada (2014). Quality assured data, national air pollution surveillance (NAPS). Ottawa. http://maps-cartes.ec.gc.ca/rnspa-naps/data.aspx?lang=en.
Erikson, K.M. and Aschner, M. (2003). Manganese neurotoxicity and glutamate-GABA interaction. Neurochem. Int., 43: 475–480.
Erikson, K.M., Dorman, D.C., Lash, L.H. and Aschner, M. (2007a). Manganese inhalation by rhesus monkeys is associated with brain regional changes in biomarkers of neurotoxicity. Toxicol. Sci., 97(2): 459–466.
Ericson, J.E., Crinella, F.M., Clarke-Stewart, K.A., Allhusen, V.D., Chan, T. and Robertson, R.T. (2007b). Prenatal manganese levels linked to childhood behavioral disinhibition. Neurotoxicol. Teratol., 29(2): 181–187.
Eum, J.H., Cheong, H.K., Ha, E.H., Ha, M., Kim, Y., Hong, Y.C., Park, H. and Chang, N. (2014). Maternal blood manganese level and birth weight: A MOCEH birth cohort study. Environ. Health, 13(1): 31-069X-13-31.
European Commission (2000). Opinion of the Scientific Committee on Food on the tolerable upper intake level of manganese. Report No. SCF/CS/NUT/UPPLEV/21.
Expert Group on Vitamins and Minerals Secretariat (EVM) (2002). Review of manganese – revised version. EVM/99/22.REVISEDAUG2002, United Kingdom.
Farina, M., Avila, D.S., da Rocha, J.B.T. and Aschner, M. (2013). Metals, oxidative stress and neurodegeneration: A focus on iron, manganese and mercury. Neurochem. Int., 62: 575–94.
FCM (2003). Water quality in distribution systems. Federation of Canadian Municipalities Available at: www.fcm.ca/Documents/reports/Infraguide/Water_Quality_in%C2%A0Distribution_System_EN.pdf
Finley, J.W. and Davis, C.D. (1999). Manganese deficiency and toxicity: Are high or low dietary amounts of manganese cause for concern? Biofactors, 10(1): 15–24. [As cited in Health Canada, 2010]
Finley, J.W. and Johnson, P.E. (1996). Manganese deficiency and excess in rodents. In: Watson, R.R. (eds.). Trace elements in laboratory rodents. Florida, CRC Press: pp. 85–102.
Finley, J.W., Johnson, P.E. and Johnson, L.K. (1994). Sex affects manganese absorption and retention by humans from a diet adequate in manganese. Am. J. Clin. Nutr., 60(6): 949–955.
Finley, J.W., Caton, J.S., Zhou, Z. and Davison, K.L. (1997). A surgical model for determination of true adsorption and biliary excretion of manganese in conscious swine fed commercial diets. J. Nutr., 127(12): 2334–2341.
Finley, J.W., Penland, J.G., Pettit, R.E. and Davis, C.D. (2003). Dietary manganese intake and type of lipid do not affect clinical or neuropsychological measures in healthy young women. J. Nutr., 133(9): 2849–2856. [As cited in Health Canada 2007.]
Fitsanakis, V.A. and Aschner, M. (2005). The importance of glutamate, glycine, and gamma-aminobutyric acid transport and regulation in manganese, mercury and lead neurotoxicity. Toxicol. Appl. Pharmacol., 204(3): 343–354.
Fitsanakis, V.A., Au, C., Erikson, K.M. and Aschner, M. (2006). The effects of manganese on glutamate, dopamine and gamma-aminobutyric acid regulation. Neurochem. Int, 48(6–7): 426–433.
Fitsanakis, V.A., Piccola, G., Marreilha dos Santos, A.P., Aschner, J.L. and Aschner, M. (2007). Putative proteins involved in manganese transport across the blood-brain barrier. Hum. Exp. Toxicol., 26(4): 295–302.
Fitsanakis, V.A., Thompson, K.N., Deery, S.E., Milatovic, D., Shihabi, Z.K., Erikson, K.M., et al. (2009). A chronic iron-deficient/high-manganese diet in rodents results in increased brain oxidative stress and behavioral deficits in the Morris water maze. Neurotox. Res., 15(2): 167–178.
Fordahl, S.C., Anderson, J.G., Cooney, P.T., Weaver, T.L., Colyer, C.L. and Erikson, K.M. (2010). Manganese exposure inhibits the clearance of extracellular GABA and influences taurine homeostasis in the striatum of developing rats. Neurotoxicology, 31(6): 639–646.
Fordahl, S., Cooney, P., Qiu, Y., Xie, G., Jia, W. and Erikson, K.M. (2012). Waterborne manganese exposure alters plasma, brain, and liver metabolites accompanied by changes in stereotypic behaviors. Neurotoxicol. Teratol., 34(1): 27–36.
Foster, M.L., Bartnikas, T.B., Johnson, L.C., Herrera, C., Pettiglio, M.A., Keene, A.M., Taylor, M.D. and Dorman, D.C. (2015). Pharmacokinetic evaluation of the equivalency of gavage, dietary, and drinking water exposure to manganese in f344 rats. Toxicol. Sci., 145(2): 244–251.
Friedman, M.J., Hill, A.S., Reiber, S.H., Valentine, R.L., Larsen, G., Young, A., Korshin, G.V. and Peng, C-Y. (2010). Assessment of Inorganics Accumulation in Drinking Water system scales and sediments. Water Research Foundation and United States Environmental Protection Agency, Denver, CO.
Furst, A. (1978). Tumorigenic effect of an organomanganese compound on F344 rats and Swiss albino mice: Brief communication. J. Natl. Cancer Inst., 60: 1171–1173. [As cited in U.S. EPA, 2008; Health Canada, 2010]
Gabelich, C.J., Gerringer, F.W., Lee, C.C., and Knocke, W.R. (2006). Sequential manganese desorption and sequestration in anthracite coal and silica sand filter media. . J. Am. Water Works Assoc., 98(5): 116–127.
Galloway, S.M., Armstrong, M.J., Reuben, C., Colman, S., Brown, B., Cannon, C., et al. (1987). Chromosome aberrations and sister chromatid exchanges in Chinese hamster ovary cells: Evaluations of 108 chemicals. Environ. Mol. Mutagen., 10(Suppl. 10): 1–175.
Garcia, S.J., Gellein, K., Syversen, T. and Aschner, M. (2006). A manganese-enhanced diet alters brain metals and transporters in the developing rat. Toxicol. Sci., 92(2): 516–525.
Garcia-Aranda, J.A., Lifshitz, F. and Wapnir, R.A. (1984). Intestinal absorption of manganese in experimental malnutrition. J. Pediatr. Gastroenterol. Nutr., 3: 602–607.
Garrick, M.D., Dolan, K.G., Horbinski, C., Ghio, A.J., Higgins, D., Porubcin, M., et al. (2003). DMT1: a mammalian transporter for multiple metals. BioMetals, 16: 41– 54. [As cited in Reany & Smith 2005.]
Gavin, C.E., Gunter, K.K. and Gunter, T.E. (1992). Mn2+ sequestration by mitochondria and inhibition of oxidative phosphorylation. Toxicol. Appl. Pharmacol., 115: 1–5. [As cited in Health Canada, 2010]
Gerber, G.B., Léonard, A. and Hantson, P. (2002). Carcinogenicity, mutagenicity and teratogenicity of manganese compounds. Crit. Rev. Oncol. Hematol., 42: 25–34.
Gibbons, R.A., Dixon, S.N., Hallis, K., Russell, A.M., Sansom, B.F. and Symonds, H.W. (1976). Manganese metabolism in cows and goats. Biochim. Biophys. Acta, 444(1): 1–10. [As cited in Davidsson et al., 1989b; Health Canada, 2010]
Gilani, S.H. and Alibhai, Y. (1990). Teratogenicity of metals to chick embryos. J. Toxicol. Environ. Health, 30: 23–31. [As cited in Gerber et al. 2002.]
Ginige, M.P., Wylie, J. and Plumb, J. (2011). Influence of biofilms on iron and manganese deposition in drinking water distribution systems. Biofouling, 27(2), 151–163.
Golub, M.S. (2012). Erratum. Neurotoxicol. Teratol., 34(1): 220.
Golub, M.S., Hogrefe, C.E., Germann, S.L., Tran, T.T., Beard, J.L., Crinella, F.M., et al. (2005). Neurobehavioral evaluation of rhesus monkey infants fed cow’s milk formula, soy formula, or soy formula with added manganese. Neurotoxicol. Teratol., 27(4): 615–627.
Gonzalez, L.E., Juknat, A.A., Venosa, A.J., Verrengia, N. and Kotler, M.L. (2008). Manganese activates the mitochondrial apoptotic pathway in rat astrocytes by modulating the expression of proteins of the Bcl-2 family. Neurochem. Int., 53(6–8): 408–415.
Government of Canada (2014). Food and Drug Regulations. Last amended on 2014-01-29; C.R.C., c. 870. Available at: http://laws.justice.gc.ca/eng/regulations/C.R.C.,_c._870
Grant, D., Blazak, W.F. and Brown, G.L. (1997). The reproductive toxicology of intravenously administered MnDPDP in the rat and rabbit. Acta Radiologica, 38: 759–769. [As cited in Health Canada, 2010]
Gray, E.L. and Laskey, J.W. (1980). Multivariate analysis of the effects of manganese on the reproductive physiology and behavior of the male house mouse. J. Toxicol. Environ. Health , 6: 861–867. [As cited in Health Canada, 2010]
Greger, J.L. (1999). Nutrition versus toxicology of manganese in humans: Evaluation of potential biomarkers. Neurotoxicology, 20(2-3): 205–212.
Gregory, D. and Carlson, K. (2003). Effect of soluble Mn concentration on oxidation kinetics. J. Am. Water Works Assoc., 95(1): 98–108.
Gruden, N. (1977). Suppression of transduodenal manganese transport by milk diet supplemented with iron. Nutr. Metab. 21(5): 305–309. [As cited in Health Canada, 2010]
Guan, H., Wang, M., Li, X., Piao, F., Li, Q., Xu, L., Kitamura, F. and Yokoyama, K. (2014). Manganese concentrations in maternal and umbilical cord blood: Related to birth size and environmental factors. Eur. J. Public Health, 24(1): 150–157.
Guilarte, T.R. (2013). Manganese neurotoxicity: new perspectives from behavioral, neuroimaging, and neuropathological studies in humans and non-human primates. Front. Aging Neurosci., 5(23): 1–10.
Gunshin, H., Allerson, C.R., Polycarpou-Schwarz, M., Rofts, A., Rogers, J.T., Kishi, F. et al. (2001). Iron-dependent regulation of the divalent metal ion transporter. FEBS Letters 509(2): 309–316. [As cited in Health Canada, 2010]
Gupta, S.K., Murthy, R.C. and Chandra, S.V. (1980). Neuromelanin in manganese-exposed primates. Toxicol. Lett., 6: 17–20.
HaMai, D. and Bondy, S.C. (2004). Oxidative basis of manganese neurotoxicity. Ann. N. Y. Acad. Sci., 1012: 129–141.
HaMai, D., Campbell, A. and Bondy, S.C. (2001). Modulation of oxidative events by multivalent manganese complexes in brain tissue. Free Radic. Biol. Med., 31(6): 763–768. [As cited in Health Canada, 2010]
HaMai, D., Rinderknecht, A.L., Guo-Sharman, K., Kleinman, M.T. and Bondy, S.C. (2006). Decreased expression of inflammation-related genes following inhalation exposure to manganese. Neurotoxicology, 27(3): 395–401.
Han, Z.J., Song, G., Cui, Y., Xia, H.F., Ma, X. (2011). Oxidative stress is implicated in arsenic-induced neural tube defects in chick embryos. Int. J. Dev. Neurosci., 29(7): 673–680.
Hargette, A., and Knocke, W.R. (2001). Assessement of fate of manganese in oxide-coated filtration systems. J. Environ. Eng., 127(2): 1132–1138.
Hauser, R.A., Zesiewicz , T.A., Rosemurgy, A.S., Martinez, C. and Olanow, C.W. (1994). Manganese intoxication and chronic liver failure. Ann. Neurol., 36(6): 871–875. [As cited in IOM, 2004, Health Canada, 2010]
Haynes, E.H., Sucharew, H., Kuhnell, P., Alden, J., Barnas, M., Wright, R.O., Parsons, P.J., Aldous, K.M., Praamsma, M.L., Beidler, C. and Dietrich, K.N. (2015). Manganese exposure and neurocognitive outcomes in rural school-age children: The Communities Actively Researching Exposure Study (Ohio, USA). Environ. Health Perspect., 123(10): 1066–1071.
Hazaradze, R.E. (1961). Hygienic background for determining the maximum permissible concentration of manganese in water basins. Gig. Sanit., 26(12): 8–14. [As cited in WHO, 1981.]
He, P., Liu, D.H. and Zhang, G.Q. (1994). Effects of high-level-manganese sewage irrigation on children's neurobehavior. Zhonghua Yu Fang Yi Xue Za Zhi, 28(4): 216–218.
Health Canada (2007a). Multi-vitamin/mineral supplements. Natural Health Products Ingredients Database, Natural Health Products Directorate, Health Canada, Ottawa, Ontario. Available at: http://webprod.hc-sc.gc.ca/nhpid-bdipsn/atReq.do?atid=multi_vitmin_suppl&lang=eng.
Health Canada (2007b). Average dietary intakes (µg/kg bw/day) of trace elements for Canadians in different age/sex groups for Total Diet Study. Bureau of Chemical Safety, Health Products and Food Branch, Health Canada, Ottawa, Ontario.
Health Canada (2009). Canadian total diet study. Available at: www.hc-sc.gc.ca/fn-an/surveill/total-diet/index-eng.php.
Health Canada (2010). Human health risk assessment for inhaled manganese. Water, Air & Climate Change Bureau, Safe Environments Programme, Health Canada. Available from: http://healthycanadians.gc.ca/publications/healthy-living-vie-saine/manganese/index-eng.php
Health Canada (2014a). Communication with A.-M. Tugulea.
Health Canada (2014b). Personal communication with R. Dabeka.
Health Canada (2015). Drinking water treatment system testing for manganese removal. Conducted under contract for Health Canada by NSF International.
Hejtmancik, M., Peters, A.C., Toft, J.D., Basaran, A., Sheridan, A. and Athey, P. (1987a). The chronic study of manganese sulfate monohydrate (CAS No. 10034-96-5) in F344 rats. Battelle's Columbus Laboratories, Columbus, Ohio. [As cited in Health Canada, 2010]
Hejtmancik, M., Peters, A.C., Toft, J.D., Dersing, R., Basaran, A., Sheridan, A., et al. (1987b). The chronic study of manganese sulfate monohydrate (CAS No. 10034-96-5) in B6C3F1 mice. Battelle's Columbus Laboratories, Columbus, Ohio. [As cited in Health Canada, 2010]
Hernandez-Bonilla, D., Schilmann, A., Montes, S., Rodriguez-Agudelo, Y., Rodriguez-Dozal, S., Solis-Vivanco, R., Rios, C. and Riojas-Rodriguez, H. (2011). Environmental exposure to manganese and motor function of children in mexico. Neurotoxicology, 32(5): 615–621.
Herzner, J.A., Parmenter, W., Page, J. and Heupler, S. (2003). Manganese control: a back to basics approach to stop customer complaints and works with unconventional processes. Proceedings of the American Water Works Association Water Quality and Technology Conference.
Hiney, J.K., Srivastava, V.K. and Dees, W.L. (2011). Manganese induces IGF-1 and cyclooxygenase-2 gene expressions in the basal hypothalamus during prepubertal female development. Toxicol. Sci., 121(2): 389–96.
Holbrook, D.J.J., Washington, M.E., Leake, H.B. and Brubaker, P.E. (1975). Studies on the evaluation of the toxicity of various salts of lead, manganese, platinum, and palladium. Environ. Health Perspect., 10: 95–101.
Holst, T.C. (2004). Large-scale biological manganese removal: from pilot study to implementation. Proceedings of the American Water Works Association Water Quality and Technology Conference.
Holzgraefe, M., Poser, W., Kijewski, H. and Beuche, W. (1986). Chronic enteral poisoning caused by potassium permanganate: A case report. J. Toxicol. Clin. Toxicol., 24(3): 235–244.
Hoyland, V.W., Knocke, W.R., Falkinham III, J.O., Pruden, A., Singh, G. (2014). Effect of drinking water treatment process parameters on biological removal of manganese from surface water. Water Res., 66: 31–39. Available at: www.epa.gov/waterscience/criteria/drinking/dwstandards2009.pdf
Hussain, S., Lipe, G.W., Slikker, W. and et al. (1997). The effects of chronic exposure of manganese on antioxidant enzymes in different regions of rat brain. Neurosci. Res. Commun., 21: 135–144.
IOM (2000). Dietary reference intakes for vitamin A, vitamin K, arsenic, boron, chromium, copper, iodine, iron, manganese, molybdenum, nickel, silicon, vanadium, and zinc. Institute of Medicine, National Academy of Sciences, National Academies Press, Washington, D.C.: pp. 394–419.
IOM (2001). Dietary reference intakes for vitamin A, vitamin K, arsenic, boron, chromium, copper, iodine, iron, manganese, molybdenum, nickel, silicon, vanadium, and zinc. Institute of Medicine, National Academy of Sciences, National Academies Press. Washington, D.C.: pp. 285–324. Available at: ww.nap.edu/catalog.php?record_id=10026.
IOM (2005). Dietary reference intakes for energy, carbohydrate, fiber, fat, fatty acids, cholesterol, protein and amino acids. Panel on Macronutrients, Panel on the Definition of Dietary Fiber, Subcommittee on Upper Reference Levels of Nutrients, Subcommittee on Interpretation and Uses of Dietary Reference Intakes, and the Standing Committee on the Scientific Evaluation of Dietary Reference Intakes Food and Nutrition Board (ed.). Institute of Medicine, National Academies Press, Washington, D.C. Available at: www.nal.usda.gov/fnic/DRI/DRI_Energy/energy_full_report.pdf.
International Manganese Institute (2014). Paris, France. Available at: www.manganese. org/index.php.
IPCS (1999). Concise international chemical assessment document 12: Manganese and its compounds. International Programme on Chemical Safety, World Health Organization. ISBN 92 4 153012 X.
Ishihara, N., Koizumi, M. and Yoshida, A. (1987). Metal concentrations in human pancreatic juice. Archives of Environmental Health, 42(6): 356–360.
Ishizuka, H., Nishida, M. and Kawada, J. (1991). Changes in stainability observed by light microscopy in the brains of ataxial mice subjected to three generations of manganese administration. Biochem. Int., 25: 677–687.
Islam, A.A., Goodwill, J.E., Bouchard, R., Tobiason, J.E., and Knocke, W.R. (2010). Characterization of filter media Mn)x(s) surfaces and Mn removal capability. J. Am. Water Works Assoc., 102(9): 71–83.
Itoh, K., Sakata, M., Watanabe, M., Aikawa, Y. and Fujii, H. (2008). The entry of manganese ions into the brain is accelerated by the activation of N-methyl-D-aspartate receptors. Abstract. Neuroscience, 154(2): 732–740.
Jarvinen, R. and Ahlström, A. (1975). Effect of the dietary manganese level on tissue manganese, iron, copper and zinc concentrations in female rats and their fetuses. Med. Biol., 53: 93–99. [As cited in ATSDR, 2012.]
Jeffcoat, S.B., Lavinder, S.R., Dye, D., and Sosebee, R. (2007). Techniques for simultaneous control of manganese and disinfection by-products at conventional water treatment facilities. Proceedings of the American Water Works Association Water Quality and Technology Conference.
Joardar, M. and Sharma, A. (1990). Comparison of clastogenicity of inorganic manganese administered in cationic and anionic forms in vivo. Mutat. Res., 240: 159–163. [As cited in Health Canada, 2010]
Johnson, P.E., Lykken, G.I. and Korynta, E.D. (1991). Absorption and biological half-life in humans of intrinsic and extrinsic 54Mn tracers from foods of plant origin. Mn. J. Nutr., 121: 711–717.
Jursa, T. and Smith, D.R. (2009). Ceruloplasmin alters the tissue disposition and neurotoxicity of manganese, but not its loading onto transferrin. Toxicol. Sci., 107(1): 182–193.
Kalea, A.Z., Harris, P.D. and Klimis-Zacas, D.J. (2005). Dietary manganese suppresses alpha1 adrenergic receptor-mediated vascular contraction. J. Nutr. Biochem., 16(1): 44–49.
Kalea, A.Z., Lamari, F.N., Theocharis, A.D., Schuschke, D.A., Karamanos, N.K. and Klimis-Zacas, D.J. (2006). Dietary manganese affects the concentration, composition and sulfation pattern of heparan sulfate glycosaminoglycans in Sprague-Dawley rat aorta. BioMetals, 19(5): 535–546.
Karim, Z. (2011). Risk assessment of dissolved trace metals in drinking water of Karachi, Pakistan. Bull. Environ. Contam. Toxicol. 86: 676–678
Karki, P., Lee, E. and Aschner, M. (2013). Manganese neurotoxicity: a focus on glutamate transporters. Ann. Occup. Environ. Med., 25(4).
Kawamura, C.L., Ikuta, H., Fukuzimi, S., Yamada, R., Tsubaki, S., Kodama, T. and Kurata S.. (1941). Intoxication by manganese in well water. Kitasato Arch. Exp. Med. 18: 145–169.
Keen, C.L., Bell, J.G. and Lönnerdal, B. (1986). The effect of age on manganese uptake and retention from milk and infant formulas in rats. J. Nutr., 116: 395–402.
Kenari, S.L.D., and Barbeau, B. (2014). Pyrolusite fluidized-bed reactor: A robust and compact process for removing manganese from groundwater. Water Res., 49: 475–483.
Kern, C. and Smith, D.R. (2011). Pre-weaning Mn exposure leads to prolonged astrocyte activation and lasting effects on the dopaminergic system in adult male rats. Synapse, 65(6): 532–544.
Kern, C.H., Stanwood, G.D. and Smith, D.R. (2010). Preweaning manganese exposure causes hyperactivity, disinhibition, and spatial learning and memory deficits associated with altered dopamine receptor and transporter levels. Synapse, 64(5): 363–378.
Khan, K., Factor-Litvak, P., Wasserman, G.A., Liu, X., Ahmed, E., Parvez, F., Slavkovich, V., Levy, D., Mey, J., van Geen, A. and Graziano, J.H. (2011). Manganese exposure from drinking water and children's classroom behavior in Bangladesh. Environ. Health Perspect., 119(10): 1501–1506.
Khan, K., Wasserman, G.A., Liu, X., Ahmed, E., Parvez, F., Slavkovich, V., Levy, D., Mey, J., van Geen, A., Graziano, J.H. and Factor-Litvak, P. (2012). Manganese exposure from drinking water and children's academic achievement. Neurotoxicology, 33(1): 91–97.
Klimis-Zacas, D. (1993). Manganese in health and disease. CRC Press: pp 54–55.
Knocke, W.R., Hoehn, R.C. and Sinsabaugh, R.L. (1987). Using alternative oxidants to remove dissolved manganese from water laden with organices. . J. Am. Water Works Assoc., 79(3): 75–79.
Knocke, W.R., Hamon, J.R., and Thompson, C.P. (1988). Soluble manganese removal on oxide-coated filter media. . J. Am. Water Works Assoc., 74(12): 65–70.
Knocke, W.R., Van Benschoten, J.E., Kearney, M., Soborski, A., and Reckhow, D.A. (1990a). Alternative oxidants for the removal of soluble manganese. American Water Works Research Foundation and American Water Works Association, Denver, Colorado.
Knocke, W.R., Occiano, S., and Hungate, R. (1990b). Removal of soluble manganese from water by oxide-coated filter media. American Water Works Research Foundation and American Water Works Association, Denver, Colorado.
Knocke, W.R., Occiano, S.C., and Hungate, R. (1991). Removal of soluble manganese by oxide-coated filter media: sorption rate and removal mechanism issues. . J. Am. Water Works Assoc., 83(8): 64–69.
Knocke, W.R., Zuransky, L., Little, J.C. and Tobiason, J.E. (2010). Adsorptive contactors for removal of soluble manganese during drinking water treatment. . J. Am. Water Works Assoc., 102(8): 64–75.
Kohl, P., Kim, A., and Charlton, N. (2002). Limitations of ozone for removal of manganese to very low levels, a comparative study. Proceedings of the American Water Works Association Water Quality and Technology Conference.
Kohl, P.M and Dixon, D. (2012). Occurrence, impacts and removal of manganese in biofiltration processes. Water Research Foundation, Denver, Colorado.
Kohl, P.M. and Medlar, S.J. (2006). Occurrence of manganese in drinking water and manganese control. American Water Research Foundation, American Water Works Association and IWA Publishing. Denver, Colorado.
Komura, J. and Sakamoto, M. (1991). Short-term oral administration of several manganese compounds in mice: Physiological and behavioral alterations caused by different forms of manganese. Bull. Environ. Contam. Toxicol., 46: 921–928. [As cited in Health Canada, 2010]
Komura, J. and Sakamoto, M. (1994). Chronic oral administration of methylcyclopentadienyl manganese tricarbonyl altered brain biogenic amines in the mouse: comparison with inorganic manganese. Toxicol. Lett., 73: 65–73.
Kondakis, X.G., Makris, N., Leotsinidis, M., Prinou, M. and Papapetropoulos, T. (1989). Possible health effects of high manganese concentration in drinking water. Arch. Environ. Health, 44(3): 175–178.
Kontur, P. and Fechter, L.D. (1985). Brain manganese, catecholamine turnover, and the development of startle in rats prenatally exposed to manganese. Teratology, 32: 1–11.
Kostial, K., Kello, D., Jugo, S., Rabar, I. and Maljković, T. (1978). Influence of age on metal metabolism and toxicity. Environ. Health Perspect., 25: 81–86.
Kostial, K., Blanusa, M., Maljković, T., Kello, D., Rabar, I. and Stara, J.F. (1989). Effect of a metal mixture in diet on the toxicokinetics and toxicity of cadmium, mercury and manganese in rats. Toxicol. Ind. Health, 5(5): 685–698. [As cited in U.S. EPA, 2004.]
Kostial, K., Blanusa, M. and Piasek, M. (2005). Regulation of manganese accumulation in perinatally exposed rat pups. J. Appl. Toxicol. 25(2): 89–93.
Koudinov, A.R. and Koudinova, N.V. (2005). Cholesterol homeostatis failure as a unifying cause of synaptic degeneration. J. Neurol. Sci., 229–230: 233–240.
Krishna, S., Dodd, C.A., Hekmatyar, S.K. and Filipov, N.M. (2014). Brain deposition and neurotoxicity of manganese in adult mice exposed via the drinking water. Arch. Toxicol., 88(1): 47–64.
Kristensson, K., Eriksson, H., Lundh, B., Plantin, O., Wachmeister, L., el Azazi, M., et al. (1986). Effects of manganese chloride on the rat developing nervous system. Acta Pharmacol. Toxicol., 59(5): 345–348. [As cited in Health Canada, 2010]
Kwik-Uribe, C. and Smith, D.R. (2006). Temporal responses in the disruption of iron regulation by manganese. J. Neurosci. Res., 83(8): 1601–1610.
Kwik-Uribe, C.L., Reaney, S., Zhu, Z. and Smith, D. (2003). Alterations in cellular IRP-dependentiron regulation by in vitro manganese exposure in undifferentiated PC12 cells. Brain Res., 973(1): 1–15.
Lai, J.C., Leung, T.K., Guest, J.F., Davison, A.N. and Lim, L. (1982). The effects of chronic manganese chloride treatment expressed as age-dependent, transient changes in rat brain synaptosomal uptake of amines. Journal of Neurochemistry, 38(3): 844–847.
Lai, J.C., Leung, T.K. and Lim, L. (1984). Differences in the neurotoxic effects of manganese during development and aging: Some observations on brain regional neurotransmitter and non-neurotransmitter metabolism in a developmental rat model of chronic manganese encephalopathy. Neurotoxicology, 5(1): 37–47.
Lai, J.C., Minski, M.J., Chan, A.W., Leung, T.K. and Lim, L. (1999). Manganese mineral interactions in brain. Neurotoxicology, 20(2–3): 433–444. [As cited in Health Canada, 2010]
Laitung, J.K. and Mercer, D.M. (1983). Manganese absorption through a burn. Burns Incl. Therm. Inj., 10(2): 145–146.
Laskey, J.W., Rehnberg, G.L., Hein, J.F. and Carter, S.D. (1982). Effects of chronic manganese (Mn3O4) exposure on selected reproductive parameters in rats. J. Toxicol. Environ. Health, 9(4): 677–687. [As cited in Health Canada, 2010;ATSDR, 2008.]
Lauwerys, R., Roels, H., Genet, P., Toussaint, G., Bouckaert, A. and de Cooman, S. (1985). Fertility of male workers exposed to mercury vapor or to manganese dust: A questionnaire study. Am. J. Ind. Med., 7(2): 171–176. [As cited in Health Canada, 2010; ATSDR, 2008.]
Lazrishvili, I.L., Shukakidze, A.A., Chkhartishvili, N.N. and Bikashvili, T.Z. (2009). Morphological changes and manganese content in the brains of rat pups subjected to subchronic poisoning with manganese chloride. Neurosci. Behav. Physiol., 39(1): 7–12.
Leavens, T.L., Rao, D., Andersen, M.E. and Dorman, D.C. (2007). Evaluating transport of manganese from olfactory mucosa to striatum by pharmacokinetic modeling. Toxicol. Sci., 97(2): 265–278.
Leblanc, A., Lapointe, S., Beaudet, A., Côté, I., Dumas, P., Labrecque, F., Lamy, C., Larochelle, J., Lepage, L., Pelletier, F. and Weber, J. (2004). Étude sur l’établissement de valeurs de référence d’éléments traces et de métaux dans le sang, le sérum et l’urine de la population de la grande région de québec. Direction toxicologie humaine, Direction risques biologiques, environnementaux et occupationnels, Institut national de santé publique du Québec. Available at: www.inspq.qc.ca/pdf/publications/289-ValeursReferenceMetaux.pdf.
Lee, B., Pine, M., Johnson, L., Rettori, V., Hiney, J.K. and Dees, W.L. (2006). Manganese acts centrally to activate reproductive hormone secretion and pubertal development in male rats. Reprod. Toxicol., 22(4): 580–585.
Lee, B., Hiney, J.K., Pine, M.D., Srivastava, V.K. and Dees, W.L. (2007). Manganese stimulates luteinizing hormone releasing hormone secretion in prepubertal female rats: hypothalamic site and mechanism of action J. Physiol., 578(3): 765–772.
Lees-Haley, P.R., Rohling, M.L. and Langhinrichsen-Rohling, J. (2006). A meta-analysis of the neuropsychological effects of occupational exposure to manganese. Clin. Neuropsychol., 20(1): 90–107.
Lei, B., Chen, L., Hao, Y., Cao, T., Zhang, X., Yu, Y., et al. (2013). Trace elements in animal-based food from Shanghai markets and associated human daily intake and uptake estimation considering bioaccessibility. Ecotoxicol. Environ. Saf., 96: 160–7.
Leung, T.K., Lai, J.C. and Lim, L. (1982). The effects of chronic manganese feeding on the ctivity of monoamine oxidase in various organs of the developing rat. Comparative Biochemistry and Physiology C, 71(2): 223–228. [As cited in U.S. EPA, 2004]
Leyva-Illades, D., Chen, P., Zogzas, C.E., Hutchens, S., Mercado, J.M., Swaim, C.D., Morrisett, R.A., Bowman, A.B., Aschner, M.and Mukhopadhyay, S. (2014). SLC30A10 is a cell surface-localized manganese efflux transporter, and parkinsonism-causing mutations block its intracellular trafficking and efflux activity. J. Neurosci., 34(42): 14079–14095.
Lin, C.C., Chen, Y.C., Su, F.C., Lin, C.M., Liao, H.F., Hwang, Y.H., Hsieh, W.S., Jeng, S.F., Su, Y.N. and Chen, P.C. (2013). In utero exposure to environmental lead and manganese and neurodevelopment at 2 years of age. Environ. Res., 123: 52–57.
Lipe, G.W., Duhart, H., Newport, G.D., Slikker, W.J. and Ali, S.F. (1999). Effect of manganese on the concentration of amino acids in different regions of the rat brain. J. Environ. Sci. Health B, 34(1): 119–132.
Liu, X., Sullivan, K.A., Madl, J.E., Legare, M. and Tjalkens, R.B. (2006). Manganese-induced neurotoxicity: the role of astroglial-derived nitric oxide in striatal interneuron degeneration. Toxicol. Sci., 91(2): 521–531.
Liu, J., Jin, L., Zhang, L., Li, Z., Wang, L., Ye, R., Zhang, Y. and Ren, A. (2013). Placental concentrations of manganese and the risk of fetal neural tube defects. J. Trace Elem. Med. Biol., 27(4): 322–325.
Ljung, K. and Vahter, M. (2007). Time to re-evaluate the guideline value for manganese in drinking water? Environ. Health Perspect., 115(11): 1533–1538.
Ljung, K.S., Kippler, M.J., Goessler, W., Grandér, G.M., Nermell, B.M. and Vahter, M.E. (2009). Maternal and early life exposure to manganese in rural Bangladesh. Environ. Sci. Technol., 43(7): 2595–2601.
Lucchini, R., Apostoli, P., Perrone, C., Placidi, D., Albini, E., Migliorati, P., Mergler, D., Sassine, M.P., Palmi, S. and Alessio, L. (1999). Long-term exposure to "low levels" of manganese oxides and neurofunctional changes in ferroalloy workers. Neurotoxicology, 20(2–3): 287–297.
Luo, Y. and Millero, F.J. (2003). Solubility of rhodochrosite (MnCO3) in NaCl solutions. J. Solution Chem., 32(5).
Malecki, E.A., Radzanowski, G.M., Radzanowski, T.J., Gallaher, D.D. and Greger, J.L. (1996). Biliary manganese excretion in conscious rats is affected by acute and chronic manganese intake but not by dietary fat. J. Nutr., 126(2): 489–498. [As cited in Health Canada, 2010]
Manitoba Conservation and Water Stewardship. (2015). Personal communication with J. Muenster.
Mann, M.A., Thomson, D., Jabari, E. and Rohe, D. (2002). Microfiltration and ultrafiltration: novel pre-treatments for iron and manganese removal from a reverse osmosis feedwater. Proceedings of the Water Quality and Technology Conference. American Water Works Association.
Martinez-Finley, E.J., Gavin, C.E., Aschner, M. and Gunter, T.E. (2013). Manganese neurotoxicity and the role of reactive oxygen species. Free Radic. Biol. Med., 62: 65–75.
McDougall, S.A., Reichel, C.M., Farley, C.M., Flesher, M.M., Der-Ghazarian, T., Cortez, A.M., et al. (2008). Postnatal manganese exposure alters dopamine transporter function in adult rats: Potential impact on nonassociative and associative processes. Neuroscience, 154(2): 848–860.
McKnight, K.F., Carlson, M., Fortin, P., and Ziesmer, C. (1993). Comparison of ozone efficiency for manganese oxidation between raw and settled water. Ozone. Sci. Eng., 15(4): 331–341
MDH (2012). Health based guidance for groundwater. Manganese. Minnesota Department of Health.
Mena, I., Horiuchi, K., Burke, K. and Cotzias, G.C. (1969). Chronic manganese poisoning: Individual susceptibility and absorption of iron. Neurology, 19(10): 1000–1006. [As cited in Health Canada, 2010]
Menezes-Filho, J.A., Novaes, C.O., Moreira, J.C., Sarcinelli, P.N. and Mergler, D. (2011). Elevated manganese and cognitive performance in school-aged children and their mothers. Environ. Res., 111(1): 156–163.
Mergler, D., Baldwin, M., Belanger, S., Larribe, F., Beuter, A., Bowler, R., Panisset, M., Edwards, R., de Geoffroy, A., Sassine, M.P. and Hudnell, K. (1999). Manganese neurotoxicity, a continuum of dysfunction: Results from a community based study. Neurotoxicology, 20(2–3): 327–342.
Merian, E., Anke, M., Ihnat, M. and Stoeppler, M. (2004). Elements and their compounds in the environment – occurence, analysis and biological relevance. 2nd ed., Weinheim, Wiley – VCH Verlag
Mettler, S., Abdelmoula, M., Hoehn, E., Schoenenberger, R., Weidler, P., and von Gunten, U. (2001). Characterization of iron and manganese precipitates from an in-situ ground water treatment plant. Groundwater, 39(6): 921–930.
Meyer-Baron, M., Schaper, M., Knapp, G., Lucchini, R., Zoni, S., Bast-Pettersen, R., Ellingsen, D.G., Thomassen, Y., He, S., Yuan, H., Niu, Q., Wang, X.L., Yang, Y.J., Iregren, A., Sjogren, B., Blond, M., Laursen, P., Netterstrom, B., Mergler, D., Bowler, R. and van Thriel, C. (2013). The neurobehavioral impact of manganese: Results and challenges obtained by a meta-analysis of individual participant data. Neurotoxicology, 36: 1–9.
Michalke, B. and Fernsebner, K. (2014). New insights into manganese toxicity and speciation. J. Trace Elem. Med. Bio., 28(2): 106–116.
Michalke, B., Halbach, S. and Nischwitz, V. (2007). Speciation and toxicological relevance of manganese in humans. J. Environ. Monit., 9(7): 650–656.
Miele, M., Serra, P.A., Esposito, G., Delogu, M.R., Migheli, R., Rocchitta, G., et al. (2000). Glutamate and catabolites of high-energy phosphates in the striatum and brainstem of young and aged rats subchronically exposed to manganese. Aging (Milano), 12(5): 393–397. [As cited in Health Canada, 2010]
Miller, E.B., Kanabrocki, E., Case, L., Graham, L., Fields, T., Oester, Y.T., et al. (1967). Non-dialyzable manganese, copper and sodium in human bile. J. Nucl. Med., 8: 891–895. [As cited in Health Canada, 2010]
Miller, S.T., Cotzias, G.C. and Evert, H.A. (1975). Control of tissue manganese: Initial absence and sudden emergence of excretion in the neonatal mouse. Am. J. Physiol., 229: 1080–1084.
Ministère du Développement durable, de l’Environnement et des Parcs du Québec (2011). Personal communication from C. Robert.
Ministère du Développement durable, de l’Environnement et des Parcs du Québec (2014). Personal communication from C. Robert.
Minoia, C., Sabbioni, E., Apostoli, P., Pietra, R., Pozzoli, L., Gallorini, M., et al. (1990). Trace element reference values in tissues from inhabitants of the European community. I. A study of 46 elements in urine, blood and serum of Italian subjects. Sci. Tot. Environ., 95: 89–105. [As cited in Health Canada, 2010]
Mirtallo, J., Canada, T., Johnson, D., Kumpf, V., Petersen, C., Sacks, G., Seres, D. and Guenter, P. (2004). Task Force for the Revision of Safe Practices for Parenteral Nutrition. Safe Practices for Parenteral. J. Parenter. Enteral. Nutr. 28, S39–S70.
Moberly, A.H., Czarnecki, L.A., Pottackal, J., Rubinstein, T., Turkel, D.J., Kass, M.D., et al. (2012). Intranasal exposure to manganese disrupts neurotransmitter release from glutamatergic synapses in the central nervous system in vivo. Neurotoxicology, 33(5): 996–1004.
Molina, R.M., Phattanarudee, S., Kim, J., Thompson, K., Wessling-Resnick, M., Maher, T.J., et al. (2011). Ingestion of Mn and Pb by rats during and after pregnancy alters iron metabolism and behavior in offspring. Neurotoxicology, 32(4): 413–422.
Montes, S., Alcaraz-Zubeldia, M., Muriel, P. et al. (2001). Striatal manganese accumulation induces changes in dopamine metabolism in the cirrhotic rat. Brain Res., 891: 123–129.
Moreno, J.A., Streifel, K.M., Sullivan, K.A., Legare, M.E. and Tjalkens, R.B. (2009a). Developmental exposure to manganese increases adult susceptibility to inflammatory activation of glia and neuronal protein nitration. Toxicol. Sci., 112(2): 405–415.
Moreno, J.A., Yeomans, E.C., Streifel, K.M., Brattin, B.L., Taylor, R.J. and Tjalkens, R.B. (2009b). Age-dependent susceptibility to manganese-induced neurological dysfunction. Toxicol. Sci., 112(2): 394–404.
Mortelmans, K., Haworth, S., Lawlor, T., Speck, W., Tainer, B. and Zeiger, E. (1986). Salmonella mutagenicity tests: II. Results from testing of 270 chemicals. Environmental Mutagenesis, 8(Suppl. 7): 1–119.
Mouchet, P. (1992). From conventional to biological of iron and manganese in France. . J. Am. Water Works Assoc., 84(4): 158–167,
Murthy, R.C., Srivastava, R.S., Gupta, S.K. and Chandra, S.V. (1980). Manganese induced testicular changes in monkeys. Exp. Pathol., 18(4): 240–244. [As cited in Health Canada, 2010]
Myers, J.E., Fine, J., Ormond-Brown, D., Fry, J., Thomson, A. and Thompson, M.L. (2009). Estimating the prevalence of clinical manganism using a cascaded screening process in a South African manganese smelter. 10th International Symposium on Neurobehavioral Methods and Effects in Environmental and Occupational Health. Neurotoxicology, 30(6): 934–940.
Na, H.K., Kim, E.H., Jung, J.H., Lee, H.H., Hyun, J.W. and Surh, Y.J. (2008). (-)-Epigallocatechin gallate induces Nrf2-mediated antioxidant enzyme expression via activation of PI3K and ERK in human mammary epithelial cells. Arch. Biochem. Biophys., 476(2): 171–177.
Nachtman, J.P., Tubben, R.E. and Commissaris, R.L. (1986). Behavioral effects of chronic manganese administration in rats: Locomotor activity studies. Neurobehav. Toxicol.Teratol., 8(6): 711–715.
Nam, J. and Kim, K. (2008). Abnormal motor function and the expression of striatal dopamine D2 receptors in manganese-treated mice. Biol. Pharm. Bull., 31(10): 1894–7.
Neal, A.P. and Guilarte, T.R. (2013). Mechanisms of lead and manganese neurotoxicity. Toxicol. Res. (Camb.), 2(2): 99–114.
New Brunswick Department of Environment (2008). New Brunswick groundwater chemistry atlas: 1994–2007. Sciences and Reporting Branch, Sciences and Planning Division, Environmental Reporting Series T2008-01.
New Brunswick Department of Health (2014). Personal communication with K. Gould and T. L. Arsenault.
Newell, G.W., Jorgenson, T.A. and Simmon, V.F. (1974). Study of mutagenic effects of manganese sulfate (FDA No. 71-71). Compound Report No. 3. U.S. Food and Drug Administration, Rockville, MD. [As cited in NTP, 1993.]
Newfoundland and Labrador Department of Environment and Conservation (2014). Personal communication from H. Khan and A. Tobin.
Newland, M.C., Cox, C, Hamada, R., Oberdorster, G. and Weiss, B. (1987). The clearance of manganese chloride in the primate. Fundam.Appl. Toxicol., 9(2): 314–328. [As cited in Health Canada, 2010]
Newland, M.C., Ceckler, T.L., Kordower, J.H. and Weiss, B. (1989). Visualizing manganese in the primate basal ganglia with magnetic resonance imaging. Experimental Neurology, 106(3): 251–258. [As cited in Health Canada, 2010]
NHMRC (2011). Australian Drinking Water Guidelines. National Health and Medical Research Council: 780–782. Available at: www.nhmrc.gov.au/guidelines/publications/eh52
Nieminski, E. and Evans, D. (1995). Pilot testing of trace metals removal with ozone at Snowbird Ski Resort. Ozone. Sci. Eng., 17: 297–309.
Nong, A., Taylor, M.D., Clewell, H.J., 3rd, Dorman, D.C. and Andersen, M.E. (2009). Manganese tissue dosimetry in rats and monkeys: accounting for dietary and inhaled Mn with physiologically based pharmacokinetic modeling. Toxicol. Sci., 108(1): 22–34.
Nong, A., Teeguarden, J.G., Clewell, H.J., 3rd, Dorman, D.C. and Andersen, M.E. (2008). Pharmacokinetic modeling of manganese in the rat IV: Assessing factors that contribute to brain accumulation during inhalation exposure. J. Toxicol. Environ. Health A, 71(7): 413–26.
Nowak, P., Bojanek, K., Szkilnik, R., Josko, J., Boron, D., Adwent, M., et al. (2011). Ontogenetic exposure of rats to pre- and post-natal manganese enhances behavioral impairments produced by perinatal 6-hydroxydopamine. Neurotox. Res., 19(4): 536–43.
Nowell, L.H. and Hoigne, J. (1987). Interaction of iron (II) and other transition metals with aqueous ozone. In: Proceedings from 8th Ozone World Congress, International Ozone Association, Norwalk, CT.
NRC (1989). Recommended dietary allowances. National Research Council (ed.). 10th edition. National Academies Press, Washington, D.C.
NSF/ANSI (2011).. Standard 372: Drinking water system components – lead content. NSF International/American National Standards Institute. NSF International, Ann Arbor, Michigan.
NSF/ANSI (2014a). Standard 42: Drinking water treatment units – aesthetic effects. NSF International/American National Standards Institute. NSF International, Ann Arbor, Michigan.
NSF/ANSI (2014b). Standard 60: Drinking water treatment chemicals – health effects. NSF International/American National Standards Institute. NSF International, Ann Arbor, Michigan.
NSF/ANSI (2014c). Standard 61: Drinking water system components – health effects. NSF International/American National Standards Institute. NSF International, Ann Arbor, Michigan.
NSF/ANSI (2014d). Standard 58: Reverse osmosis drinking water treatment systems. NSF International/American National Standards Institute. NSF International, Ann Arbor, Michigan.
NSF/ANSI (2014e). Standard 44: Cation exchange water softeners. NSF International/American National Standards Institute. NSF International, Ann Arbor, Michigan.
NTIS (1973). Teratologic evaluation of FDA 71-71 (manganese sulfate, monohydrate). PB 223-813. U.S. Department of Commerce, Springfield, VA. [As cited in NTP, 1993.]
NTP (1993). Toxicology and carcinogenesis studies of manganese (II) sulfate monohydrate (CAS No. 10034-96-5) in F344/N rats and B6C3F1 mice (feed studies). National Toxicology Program. Report No. TR-428. Available at: http://ntp.niehs.nih.gov/?objectid=0709D83B-9FDB-28AC-789C940D7855E09C.
O’Brien, W.J., Lee, E., Freeman, S., Shorney, H., Long, B., and Elder, D. (1996). Manganese oxidation and removal by ozone and polymer-assisted filtration. Proceedings 1996 AWWA Water Quality Technology Conference. Boston, MA.
Oberly, T.J., Piper, C.E. and McDonald, D.S. (1982). Mutagenicity of metal salts in the L5178Y mouse lymphoma assay. J. Toxicol. Environ. Health, 9: 367–376. [As cited in Health Canada, 2010]
OECD (2007). OECD Guideline for the Testing of Chemicals. Developmental Neurotoxicity Study (TG 426). Available at: www.oecd-ilibrary.org/environment/test-no-426-developmental-neurotoxicity-study_9789264067394-en;jsessionid=8a0ycpkew639.x-oecd-live-02.
Ohishi, T., Wang, L., Akane, H., Shiraki, A., Goto, K., Ikarashi, Y., et al. (2012). Reversible aberration of neurogenesis affecting late-stage differentiation in the hippocampal dentate gyrus of rat offspring after maternal exposure to manganese chloride. Reprod. Toxicol., 34(3): 408–419.
Öner, G. and Sentürk, U.K. (1995). Reversibility of manganese-induced learning defect in rats. Food Chemistry and Toxicology, 33(7): 559–563.
Ontario Ministry of the Environment (2014). Personal communication with S. Deshpande.
Oszlanczi, G., Vezer, T., Sarközi, L., Horvath, E., Szabo, A., Konya, Z., et al. (2010). Metal deposition and functional neurotoxicity in rats after 3-6 weeks nasal exposure by two physicochemical forms of manganese. Environ Toxicol Pharmacol., 30(2): 121–126.
Oulhote, Y., Mergler, D., Barbeau, B., Bellinger, D.C., Bouffard, T., Brodeur, M.E., Saint-Amour, D., Legrand, M., Sauve, S. and Bouchard, M.F. (2014). Neurobehavioral function in school-age children exposed to manganese in drinking water. Environ. Health Perspect., 122(12): 1343–1350.
Ouvrard, S., Simonnot, M-O., Sardin, M. (2002). Reactive behavior of natural manganese oxides toward the adsorption of phosphate and arsenate. Ind. Eng. Chem. Res., 41, 2785–2791.
Pagano, D.A. and Zeiger, E. (1992). Conditions for detecting the mutagenicity of divalent metals in Salmonella typhimurium. Environ. Mol. Mutagen., 19(2): 139–146. [As cited in Health Canada, 2010]
Paillard, , H., Legube, B., Bourbigot, M.M. and Lefebvre, E. (1989). Iron and manganese removal with ozonation in the presence of humic substances. Ozone-Sci. Eng., 11(1): 93–99.
Pal, P.K., Samii, A. and Calne, D.B. (1999). Manganese neurotoxicity: A review of clinical features, imaging and pathology. Neurotoxicology, 20(2–3): 227–238. [As cited in Health Canada, 2010]
Pappas, B.A., Zhang, D., Davidson, C.M., Crowder, T., Park, G.A. and Fortin, T. (1997). Perinatal manganese exposure: Behavioral, neurochemical, and histopathological effects in the rat. Neurotoxicol. Teratol., 19(1): 17–25.
Park, N.H., Park, J.K., Choi, Y., Yoo, C.I., Lee, C.R., Lee, H., et al. (2003). Whole blood manganese correlates with high signal intensities on T1-weighted MRI in patients with liver cirrhosis. Neurotoxicology, 24(6): 909–915. [As cited in Health Canada, 2010]
Park, J.D., Chung, Y.H., Kim, C.Y., Ha, C.S., Yang, S.O., Khang, H.S., et al. (2007). Comparison of high MRI T1 signals with manganese concentration in brains of cynomolgus monkeys after 8 months of stainless steel welding-fume exposure. Inhal. Toxicol., 19(11): 965–971.
Parry, J.M. (1977). The use of yeast cultures for the detection of environmental mutagens using a fluctuation test. Mutat. Res., 46: 165–176. [As cited in NTP, 1993.]
Peneder, T.M., Scholze, P., Berger, M.L., Reither, H., Heinze, G., Bertl, J., et al. (2011). Chronic exposure to manganese decreases striatal dopamine turnover in human alpha-synuclein transgenic mice. Neuroscience, 180: 280–92.
Peng, C-Y., Korshin, G.V. (2011). Speciation of trace inorganic contaminants in corrosion scales and deposits formed in drinking water distribution systems. Water Res., 45: 5553–5563.
Pine, M., Lee, B., Dearth, R., Hiney, J.K. and Dees, W.L. (2005). Manganese acts centrally to stimulate luteinizing hormone secretion: a potential influence on female pubertal development. Toxicol. Sci., 85: 880–885.
Pollack, S., George, J.N. and Reba, R.C. (1965). The absorption of nonferrous metals in iron deficiency. J. Clin. Invest., 44: 1470–1473.
Ponnapakkam, T.P., Bailey, K.S., Graves, K.A. and Iszard, M.B. (2003a). Assessment of male reproductive system in the CD-1 mice following oral manganese exposure. Reprod. Toxicol., 17(5): 547–551. [As cited in Health Canada, 2010]
Ponnapakkam, T., Iszard, M. and Henry-Sam, G. (2003b). Effects of oral administration of manganese on the kidneys and urinary bladder of Sprague-Dawley rats. Int. J. Toxicol., 22(3): 227–232. [As cited in Health Canada, 2010]
Ponnapakkam, T.P., Sam, G.H. and Iszard, M.B. (2003c). Histopathological changes in the testis of the Sprague Dawley rat following orally administered manganese. Bull. Environ. Contam. Toxicol., 71(6): 1151–1157. [As cited in Health Canada, 2010]
Prestifilippo, J.P., Fernandez-Solari, J., Mohn, C., De Laurentiis, A., McCann, S.M., Dees, W., et al. (2007). Effect of manganese on luteinizing hormone-releasing hormone secretion in adult male rats. Toxicol. Sci., 97(1): 75–80.
Prestifilippo, J.P., Fernandez-Solari, J., De Laurentiis, A., Mohn, C.E., de la Cal, C., Reynoso, R., et al. (2008). Acute effect of manganese on hypothalamic luteinizing hormone releasing hormone secretion in adult male rats: involvement of specific neurotransmitter systems. Toxicol. Sci., 105(2): 295–302.
Racette, B.A., Aschner, M., Guilarte, T.R., Dydak, U., Criswell, S.R. and Zheng, W. (2012). Pathophysiology of manganese-associated neurotoxicity. Neurotoxicology, 33(4): 881–886.
Raiten, D.J., Talbot, J.M. and Waters, J.H. (1998). Assessment of nutrient requirements for infant formulas. Life Sciences Research Office, American Society for Nutritional Sciences (ed.). Volume 128 (No. 11S): 2059S–2294S, Bethesda, Maryland, http://jn.nutrition.org/content/128/11/suppl/DC1.
Ranasinghe, J.G.S., Liu, M., Sakakibara, Y. et al. (2000). Manganese administration induces the increased production of dopamine sulfate and depletion of dopamine in Sprague-Dawley rats. J. Biochem. (Tokyo), 128: 477–480.
Rasmuson, A. (1985). Mutagenic effects of some water-soluble metal compounds in a somatic eye-color test system in Drosophila melanogaster. Mutat. Res., 157: 157–162. [As cited in Assem et al., 2011].
Reaney, S.H. and Smith, D.R. (2005). Manganese oxidation state mediates toxicity in PC12 cells. Toxicol. Appl. Pharmacol., 205(3): 271–281.
Reaney, S.H., Bench, G. and Smith, D.R. (2006). Brain accumulation and toxicity of Mn(II) and Mn(III) exposures. Toxicol. Sci., 93(1): 114–124.
Reaney, S.H., Kwik-Uribe, C.L. and Smith, D.R. (2002). Manganese oxidation state and its implications for toxicity. Chemical Research in Toxicology, 15(9): 1119–1126.
Rehnberg, G.L., Hein, J.F., Carter, S.D., Linko, R.S. and Laskey, J.W. (1982). Chronic ingestion of Mn3O4 by young rats: Tissue accumulation and distribution of manganese in two generations:. J. Toxicol. Environ. Health, 9(2): 175–188. [As cited in Health Canada, 2010]
Reichel, C.M., Wacan, J.J., Farley, C.M., Stanley, B.J., Crawford, C.A. and McDougall, S.A. (2006). Postnatal manganese exposure attenuates cocaine-induced locomotor activity and reduces dopamine transporters in adult male rats. Neurotoxicol. Teratol., 28(3): 323–332.
Rink, S.M., Ardoino, G., Queirolo, E.I., Cicariello, D., Manay, N. and Kordas, K. (2014). Associations between hair manganese levels and cognitive, language, and motor development in preschool children from Montevideo, Uruguay. Arch. Environ. Occup. Health, 69(1): 46–54.
Riojas-Rodriguez, H., Solis-Vivanco, R., Schilmann, A., Montes, S., Rodriguez, S., Rios, C. and Rodriguez-Agudelo, Y. (2010). Intellectual function in Mexican children living in a mining area and environmentally exposed to manganese. Environ. Health Perspect., 118(10): 1465–1470.
Roels, H.A., Ghyselen, P., Buchet, J.P., Ceulemans, E. and Lauwerys, R.R. (1992). Assessment of the permissible exposure level to manganese in workers exposed to manganese dioxide dust. Br. J. Ind. Med., 49(1): 25–34.
Roels, H.A., Meiers, G., Delos, M., Ortega, I., Lauwerys, R.R., Buchet, J.P., et al. (1997). Influence of the route of administration and the chemical form (MnCl2, MnO2) on the absorption and cerebral distribution of manganese in rats. Arch. Toxicol., 71(4): 223–230.
Roels, H.A., Bowler, R.M., Kim, Y., Claus Henn, B., Mergler, D., Hoet, P., Gocheva, V.V., Bellinger, D.C., Wright, R.O., Harris, M.G., Chang, Y., Bouchard, M.F., Riojas-Rodriguez, H., Menezes-Filho, J.A. and Tellez-Rojo, M.M. (2012). Manganese exposure and cognitive deficits: A growing concern for manganese neurotoxicity. Neurotoxicology, 33(4): 872–880.
Roth, J.A. (2006). Homeostatic and toxic mechanisms regulating manganese uptake, retention, and elimination. Biol. Res., 39(1): 45–57.
Roth, J.A., Li, Z., Sridhar, S. and Khoshbouei, H. (2013). The effect of manganese on dopamine toxicity and dopamine tranposter (DAT) in control and DAT transfected HEK cells. Neurotoxicology, 35: 121–8.
Rumsby, P., Clegg, H., Jonsson, J., Benson, V., Harman, M., Doyle, T., Rushton, L., Warwick, P., Wilkinson, D. and Prepared for the Drinking Water Inspectorate (2014). Speciation of manganese in drinking water. National Centre for Environmental Toxicology, UC9780.
Ruoff, W.L. (1995). Relative bioavailability of manganese ingested in food or water. In: Proceedings of the Workshop on the Bioavailability and Oral Toxicity of Manganese. Sponsored by the U.S. Environmental Protection Agency, Cincinnati, OH, August 30-31, 1994. [cited in U.S. EPA, 2002]
Sahabi, D. M., Takeda, M., Suzuki, I., and Koizumi, J. (2009). Removal of Mn2+ from water by “aged” biofilter media: The role of catalytic oxides layers. J. Biosci. Bioeng., 107(2): 151–157,
Sahni V, Léger Y, Panaro L, Allen M, Giffin S, Fury D, Hamm N. (2007). Case report: a metabolic disorder presenting as pediatric manganism. Environ. Health Perspect., 115(12): 1776–1779.
Sain, A.E, Griffin, A., and Dietrich, A.M (2014). Assessing taste and visual perception of Mn(II) and Mn(IV). . J. Am. Water Works Assoc., 106(1): 32–40.
Salehi, F., Krewski, D., Mergler, D., Normandin, L., Kennedy, G., Philippe, S., et al. (2003). Bioaccumulation and locomotor effects of manganese phosphate/sulfate mixture in Sprague-Dawley rats following subchronic (90 day) inhalation exposure. Toxicol. Appl. Pharmacol., 191(3): 264–271.
Salehi, F., Normandin, L., Krewski, D., Kennedy, G., Philippe, S. and Zayed, J. (2006). Neuropathology, tremor and electromyogram in rats exposed to manganese phosphate/sulfate mixture. J. Appl. Toxicol., 26(5): 419–426.
Sanchez, B., Casalots-Casado, J., Quintana, S., Arroyo, A., Martin-Fumado, C. and Galtes, I. (2012). Fatal manganese intoxication due to an error in the elaboration of epsom salts for a liver cleansing diet. Forensic Sci. Int., 223(1–3): e1–4.
Sánchez, D.J., Domingo, J.L., Llobet, J.M. and Keen, C.L. (1993). Maternal and developmental toxicity of manganese in the mouse. Toxicology Letters, 69(1): 45–52. [As cited in Health Canada, 2010]
Sandstrom, B., Davidsson, L., Cederblad, A., Eriksson, R. and Lonnerdal, B. (1986). Manganese absorption and metabolism in man. Acta Pharmacol. Toxicol., 59(Suppl 7): 60–62. [As cited in Health Canada, 2010]
Santamaria, A.B. (2008). Manganese exposure, essentiality & toxicity. Indian J. Med. Res., 128(4): 484–500.
Saric, M. (1986). Manganese. In: Frieberg, L., Nordberg, G.F. & Vouk, V.B. (eds.). Handbook on the Toxicology of Metals. Volume II: Specific Metals. 2nd ed. Amsterdam, Elsevier Science Publishers BV (354–386). [As cited in Geber et al., 2002, Merian et al., 2004.]
Saskatchewan Water Security Agency (2014). Personal communication from S. Ferris.
Saudin, F., Gelas, P. and Bouletreau, P. (1988). Trace elements in artificial nutrition. art and practice. Ann. Fr. Anesth. Reanim., 7(4): 320–332.
Schneider, C., Johns, P., and Huehmer, R. (2001). Microfiltration for removal of manganese from surface water. AWWA 2001 Annual Conference Proceedings, Washington, D.C.
Schock, M.R. (2005). Distribution systems as reservoirs and reactors for inorganic contaminants. Chapter 6 in: Distribution system water quality challenges in the 21st century – a strategic guide. M.J. Macphee (ed.). American Water Works Association, Denver, Colorado.
Schock, M.R. and Holm, T.R. (2003). Are we monitoring in the right places for metals and radionuclides? Journal of the New England Water Works Association, 141(2): 102.
Schock, M.R., Cantor, A.F., Triantafyllidou, S., Desantis, M.K., Scheckel, K.G. (2014). Importance of pipe deposits to Lead and Copper Rule compliance. J. Am. Water Works Assoc., 106(7), E336–E349.
Schroeder, H.A., Balassa, J.J. and Tipton, I.H. (1966). Essential trace metals in man: Manganese. A study in homeostasis. J. Chronic Dis., 19(5): 545–571. [As cited in Health Canada, 2010]
Schroeter, J.D., Nong, A., Yoon, M., Taylor, M.D., Dorman, D.C., Andersen, M.E., et al. (2011). Analysis of manganese tracer kinetics and target tissue dosimetry in monkeys and humans with multi-route physiologically based pharmacokinetic models. Toxicol. Sci., 120(2): 481–498.
Schroeter, J.D., Dorman, D.C., Yoon, M., Nong, A., Taylor, M.D., Andersen, M.E., et al. (2012). Application of a multi-route physiologically based pharmacokinetic model for manganese to evaluate dose-dependent neurological effects in monkeys. Toxicol. Sci., 129(2): 432–446.
SCF (2003). Report of the scientific committee on food on the revision of essential requirements of infant formulae and follow-on formulae. Scientific Committee on Food, Health and Consumers Directorate-General, European Commission, SCF/CS/NUT/IF/65 Final, Brussels, Belgium.
Seel, N.M. (2012). Basal Ganglia. In: Seel, N.M. (Ed.) Encyclopedia of the Sciences of Learning. Springer US. Available at: http://dx.doi.org/10.1007/978-1-4419-1428-6_2043.
Sentürk, U.K. and Öner, G. (1996). The effect of manganese-induced hypercholesterolemia on learning in rats. Biol. Trace Elem. Res., 51( 3): 249–257.
Sharma, R. and Pervez, S. (2005). Toxic metals status in human blood and breast milk samples in an integrated steel plant environment in Central India. Environ. Geochem. Health, 27(1): 39–45.
Shi, X.Q., Yan, W., Wang, K.Y., Fan, Q.Y. and Zou, Y. (2012). Protective effects of dietary fibre against manganese-induced neurobehavioral aberrations in rats. Arh. Hig. Rada. Toksikol., 63(3): 263–270.
Shukakidze, A.A., Lazriev, I.L. and Mitagvariya, N. (2003). Behavioral impairments in acute and chronic manganese poisoning in white rats. Neurosci. Behav. Physiol., 33(3): 263–267.
Shukakidze, A.A., Lazriev, I.L., Khetsuriani, R.G. and Bikashvili, T.Z. (2002). Changes in neuroglial ultrastructure in various parts of the rat brain during manganese chloride poisoning. Neurosci. Behav. Physiol., 32(6): 561–566.
Shukla, G.S., Singh, S. and Chandra, S.V. (1978). The interaction between manganese and ethanol in rats. Acta Pharmacol. Toxicol., 43(5): 354–362.
Shukla, G.S., Chandra, S.V. and Seth, P.K. (1976). Effect of manganese on the levels of DNA, RNA, DNase and RNase in cerebrum, cerebellum and rest of brain regions of rat. Acta Pharmacol. Toxicol., 39(5): 562–569. [As cited in Health Canada, 2010]
Shukla, G.S., Dubey, M.P. and Chandra, S.V. (1980). Manganese-induced biochemical changes in growing versus adult rats. Archives of Environmental Contamination & Toxicology, 9(4): 383–391. [As cited in Health Canada, 2010]
Shumay, E., Fowler, J.S. and Volkow, N.D. (2010). Genomic features of the human dopamine transporter gene and its potential epigenetic states: Implications for phenotypic diversity. PLOS ONE, 5(6): e11067.
Sialelli, J., Urquhart, G.J., Davidson, C.M. and Hursthouse, A.S. (2010). Use of a physiologically based extraction test to estimate the human bioaccessibility of potentially toxic elements in urban soils from the city of Glasgow, UK. Environ. Geochem. Health, 32(6): 517–27.
Siddappa, A.J., Rao, R.B., Wobken, J.D., Leibold, E.A., Connor, J.R. and Georgieff, M.K. (2002). Developmental changes in the expression of iron regulatory proteins and iron transport proteins in the perinatal rat brain. J. Neurosci. Res., 68(6): 761–775.
Sidoryk-Wegrzynowicz, M., Lee, E., Albrecht, J. and Aschner, M. (2009). Manganese disrupts astrocyte glutamine transporter expression and function. J. Neurochem., 110(3): 822–830.
Sidoryk-Wegrzynowicz, M. and Aschner, M. (2013a). Role of astrocytes in manganese mediated neurotoxicity. BMC Pharmacol. Toxicol., 14(23).
Sidoryk-Wegrzynowicz, M. and Aschner, M. (2013b). Manganese toxicity in the central nervous system: the glutamine/glutamate-gamma-aminobutyric acid cycle. J. Intern. Med., 273: 466–477.
Singh, I. (1984). Induction of gene conversion and reverse mutation by manganese sulphate and nickel sulphate in Saccharomyces cerevisiae. Mutat. Res. 137(1): 47–49. [As cited in Health Canada, 2010]
Sly, L.I., Hodgkinson, M.C., Arunpairojana, V. 1990. Deposition of manganese in drinking water distribution systems. Appl. Environ. Microbiol., 56(3), 628–639.
Smyth, H.F., Carpenter, C.P., Weil, C.S., Pozzani, U.C., Striegal, J.A. and Nycum, J.S. (1969). Range-finding toxicity data: List VII. AIHA, 30: 470–476. [As cited in U.S. EPA, 2004, WHO, 2011.]
Sommerfield, E.O. (1999). Iron and manganese removal handbook. American Water Works Association. Denver, Colorado.
Spadoni, F., Stefani, A., Morello, M., Giacomini, P. and Sancesario, G. (2000). Selective vulnerability of pallidal neurons in the early phases of manganese intoxication. Exp. Brain Res., 135(4): 544–551.
Stokes, P.M., Campbell, P.G.C., Schroeder, W.H., Trick, C., France, R.L., Puckett, K.J., LaZerte, B., Speyer, M., Hanna, J.E. and Donaldson, J. (1988). Manganese in the Canadian environment. NRCC (ed.).
Subhash, M.N. and Padmashree, T.S. (1991). Effect of manganese on biogenic amine metabolism in regions of the rat brain. Food Chem. Toxicol., 29: 579–82.
Szakmáry, E., Ungváry, G., Hudák, A., Naray, M., Tatrai, E., Szeberenyi, S., et al. (1995). Developmental effect of manganese in rat and rabbit. Central European Journal of Occupational and Environmental Medicine, 1(2): 149–159. [As cited in WHO, 1999.]
Takacs, S., Szabo, A., Oszlanczi, G., Paulik, E. and Papp, A. (2012). A pilot study with simultaneous recording of changes in motility and cortical electrical activity of rats during four weeks of oral manganese exposure. Int. J. Environ. Health Res., 22(4): 331–9.
Takeda, A., Sawashita, J. and Okada, S. (1995). Biological half-lives of zinc and manganese in rat brain. Brain Research, 695(1): 53–58. [As cited in Health Canada, 2007.]
Tapin, D., Kennedy, G., Lambert, J. and Zayed, J. (2006). Bioaccumulation and locomotor effects of manganese sulfate in Sprague-Dawley rats following subchronic (90 days) inhalation exposure. Toxicol. Appl. Pharmacol., 211(2): 166–174.
Taylor, M.D., Clewell, H.J. 3rd, Andersen, M.E., Schroeter, J.D., Yoon, M., Keene, A.M., and Dorman, D.C. (2012). Update on a pharmacokinetic-centric alternative tier II program for MMT-Part II: Physiologically based pharmacokinetic modeling and manganese risk assessment. J. Toxicol., Article ID 791431.
Taylor, M.D., Erikson, K.M., Dobson, A.W., Fitsanakis, V.A., Dorman, D.C. and Aschner, M. (2006). Effects of inhaled manganese on biomarkers of oxidative stress in the rat brain. Neurotoxicology, 27(5): 788–797.
Teeguarden, J.G., Dorman, D.C., Covington, T.R., Clewell, H.J. 3rd and Andersen, M.E. (2007a). Pharmacokinetic modeling of manganese. I. Dose dependencies of uptake and elimination. J. Toxicol. Environ. Health A., 70(18): 1493–1504.
Teeguarden, J.G., Dorman, D.C., Nong, A., Covington, T.R., Clewell, H.J. 3rd and Andersen, M.E. (2007b). Pharmacokinetic modeling of manganese. II. Hepatic processing after ingestion and inhalation. J. Toxicol. Environ. Health A, 70(18): 1505–1514.
Teeguarden, J.G., Gearhart, J., Clewell, H.J. 3rd, Covington, T.R., Nong, A. and Andersen, M.E. (2007c). Pharmacokinetic modeling of manganese. III. Physiological approaches accounting for background and tracer kinetics. J. Toxicol. Environ. Health A., 70(18): 1515–1526.
Thompson, K.J., Molina, R.M. and Donaghey, T.E.A. (2011). Manganese uptake and distribution in the brain after methyl bromide-induced lesions in the olfactory epithelia. Toxicol. Sci., 120(1): 163–72.
Tjalkens, R.B., Zoran, M.J., Mohl, B. and Barhoumi, R. (2006). Manganese suppresses ATP-dependent intercellular calcium waves in astrocyte networks through alteration of mitochondrial and endoplasmic reticulum calcium dynamics. Brain Res., 1113(1): 210–219.
Tobiason, J.E., Knocke, W.R., Islam, A.A., and Goodwill, J.E. (2007). Control of periodically elevated raw water manganese with oxide coated media. Proceedings of the American Water Works Association Water Quality Technology Conference.
Tobiason, J.E., Islam, A.A., Knocke, W.R., Goodwill, J., Hargette, P., Bouchard, R., and Zuravnsky, L. (2008). Characterization and performance of filter media for manganese control. American Water Works Research Foundation, Denver, Colorado.
Torrente, M., Colomina, M.T. and Domingo, J.L. (2005a). Metal concentrations in hair and cognitive assessment in an adolescent population. Biol. Trace Elem. Res., 104(3): 215–221.
Torrente, M., Colomina, M.T. and Domingo, J.L. (2005b). Behavioral effects of adult rats concurrently exposed to high doses of oral manganese and restraint stress. Toxicology, 211(1–2): 59–69.
Torres-Agustin, R., Rodriguez-Agudelo, Y., Schilmann, A., Solis-Vivanco, R., Montes, S., Riojas-Rodriguez, H., et al. (2013). Effect of environmental manganese exposure on verbal learning and memory in Mexican children. Environ. Res., 121: 39–44.
Tran, T.T., Chowanadisai, W., Crinella, F.M., Chicz-Demet, A. and Lonnerdal, B. (2002a). Effect of high dietary manganese intake of neonatal rats on tissue mineral accumulation, striatal dopamine levels, and neurodevelopmental status. Neurotoxicology 23(4–5): 635–643.
Tran, T.T., Chowanadisai, W., Lonnerdal, B., Le, L., Parker, M., Chicz-Demet, A., et al. (2002b). Effects of neonatal dietary manganese exposure on brain dopamine levels and neurocognitive functions. Neurotoxicology 23(4–5): 645–651.
Treinen, K.A., Gray, T.J.B. and Blazak, W.F. (1995). Developmental toxicity of mangafodipir trisodium and manganese chloride in Sprague-Dawley rats. Teratology, 52: 109–115. [As cited in Health Canada, 2010]
Tsuda, H. and Kato, K. (1977). Chromosomal aberrations and morphological transformation in hamster embryonic cells treated with potassium dichromate in vitro. Mutat. Res., 46: 87–94. [As cited in ATSDR, 2008.]
Tuschl, K., Mills, P.B. and Clayton, P.T. (2013). Manganese and the brain. Int. Rev. Neurobiol., 110: 277–312.
Ueda, F., Raja, K.B., Simpson, R.J., Trowbridge, I.S. and Bradbury, M.W. (1993). Rate of 59Fe uptake into brain and cerebrospinal fluid and the influence thereon of antibodies against the transferrin receptor. J. Neurochem., 60(1): 106–113. [Cité dans Santé Canada, 2010]
U.S. EPA (1979). National Secondary Drinking Water Regulations; Final Rule. Federal Register 44(140): 42195–42202.
U.S. EPA (1986). Guidelines for the health risk assessment of chemical mixtures. U. S. Environmental Protection Agency. Federal Register 51(185): 34014–34025.
U.S. EPA (1988). Recommendations for and documentation of biological values for use in risk assessment. U.S. Environmental Protection Agency. Office of Health and Environmental Assessment, Environmental Criteria and Assessment Office, Cincinnati, Ohio.
U.S. EPA (1994a). Method 200.7: Determination of metals and trace elements in water and wastes by inductively coupled plasma-atomic emission spectrometry. Rev 4.4. Environment Monitoring Systems Laboratories. Office of Research and Development, Cincinnati, Ohio 45268.
U.S. EPA (1994b). Method 200.8, Revision 5.4: Determination of trace elements in waters and wastes by Inductively Coupled Plasma-Mass Spectrometry. Environment Monitoring Systems Laboratories. Office of Research and Development, Cincinnati, Ohio 45268
U.S. EPA (1994c). Method 200.9, Revision 2.2: Determination of trace elements by stabilized temperature graphite furnace atomic adsorption. Environment Monitoring Systems Laboratories. Office of Research and Development, Cincinnati, Ohio 45268
U.S. EPA (1994d). Method 218.6, Revision 3.3: Determination of dissolved hexavalent chromium in drinking water, groundwater and industrial wastewater effluents by ion chromatography. Environment Monitoring Systems Laboratories. Office of Research and Development, Cincinnati, OH.
U.S. EPA (1999). Guidelines for carcinogen risk assessment. National Center for Environmental Assessment, Risk Assessment Forum, U.S. Environmental Protection Agency. Washington, D.C. NCEA-F-0644. July 1999. Available at: www.epa.gov/ncea/raf/car2sab.htm
U.S. EPA (2002). Manganese (CASRN 7439-96-5). Integrated Risk Information System (IRIS). Last revision: 1996 ed., National Center for Environmental Assessment (NCEA), U.S. Environmental Protection Agency. Available at: www.epa.gov/ncea/iris/subst/0373.htm. Last revision: 2002.
U.S. EPA (2003). Method 200.5. Determination of trace elements in drinking water by axially viewed inductively coupled plasma – atomic emission spectrometry. National exposure research laboratory. Office of research and development. Ohio 45268. EPA 600/R-06/115
U.S. EPA (2004). Drinking water health advisory for manganese. Office of Water, Health and Ecological Criteria Division, EPA-822-R-04-003, Washington. Available at: www.epa.gov/safewater/ccl/pdfs/reg_determine1/support_cc1_magnese_dwreport.pdf
U.S. EPA (2006). Inorganic contaminant accumulation in potable water distribution systems. Office of Water, U.S. Environmental Protection Agency, Washington, D.C. Available at: www.epa.gov/ogwdw/disinfection/tcr/pdfs/issuepaper_tcr_inorganiccontaminantaccumulation.pdf
U.S. EPA (2009). 2009 edition of the drinking water standards and health advisories. United States EPA 822-R-09-011, Office of Water, U.S .Environmental Protection Agency, Washington, D.C. Available at: www.epa.gov/waterscience/criteria/drinking/dwstandards2009.pdf
U.S. EPA (2010). Technical basis for the lowest concentration minimum reporting level (LCMRL) calculation. Office of Water (MLK 140) EPA 815-R-11-001 December 2010 http://water.epa.gov/drink
U.S. EPA (2012). Federal Registry Part II. Revisions of the Unregulated Contaminant Monitoring Regulation (UCMR 3) for public water systems; Final Rule. Vol 77, No 85. May 2, 2012. U.S.Environmental Protection Agency, Washington, D.C.
Valencia, R., Mason, J.M., Woodruff, R.C. and Zimmering, S. (1985). Chemical mutagenesis testing in Drosophila. III. Results of 48 coded compounds tested for the National Toxicology Program. Environ. Mutagen., 7(3): 325–348.
Vezer, T., Kurunczi, A., Naray, M., Papp, A. and Nagymajtényi, L. (2007). Behavioral effects of subchronic inorganic manganese exposure in rats. Am. J. Ind. Med., 50(11): 841–852.
Vezer, T., Papp, A., Z, H., Varga, M., Naray, M. and Nagymajtényi, L. (2005). Behavioral and neurotoxicological effects of subchronic manganese exposure in rats. Environ. Toxicol. Pharmacol, 19: 797–810.
Vieregge, P., Heinzow, B., Korf, G., Teichert, H.M., Schleifenbaum, P. and Mosinger, H.U. (1995). Long term exposure to manganese in rural well water has no neurological effects. Can. J. Neurol. Sci., 22: 286–289.
Vreeburg, J. (2010). Discolouration in Drinking Water Systems: The Role of Particles Clarified. London: IWA Publishing.
Vreeburg, J.H.G., Boxall, J.B. (2007). Discolouration in potable water distribution systems: A review. Water Res., 41: 519–529.
Vreeburg, J.H.G., Schippers, D., Verberk, J.Q.J.C., van Dijk, J.C. (2008). Impact of particles on sediment accumulation in a drinking water distribution system. Water Res., 42, 4233–4242.
Wallace, L. and Slonecker, T. (1997). Ambient air concentrations of fine (PM25) manganese in U.S. national parks and in California and Canadian cities: The possible impact of adding MMT to unleaded gasoline. J.Air Waste Manage. Assoc., 47(6): 642–652.
Walsh, M.P. (2007). The global experience with lead in gasoline and the lessons we should apply to the use of MMT. Am. J. Ind. Med., 50(11): 853–860.
Wang, L., Ohishi, T., Shiraki, A., Morita, R., Akane, H., Ikarashi, Y., et al. (2012). Developmental exposure to manganese chloride induces sustained aberration of neurogenesis in the hippocampal dentate gyrus of mice. Toxicol. Sci., 127(2): 508–21.
Ward, J.M. (1980). The carcinogenicity of ethylene dichloride in Osborne-Mendel rats and B6C3F1 mice. In: Ames, B., Infante, P. and Reitz, R. (eds.). Ethylene dichloride: A potential health risk? Banbury Report No. 5. Cold Spring Harbor Laboratory, Cold Spring Harbor, New York, New York. pp. 35–53.
Wasserman, G.A., Liu, X., Parvez, F., Ahsan, H., Levy, D., Factor-Litvak, P., Kline, J., van Geen, A., Slavkovich, V., LoIacono, N.J., Cheng, Z., Zheng, Y. and Graziano, J.H. (2006). Water manganese exposure and children's intellectual function in Araihazar, Bangladesh. Environ. Health Perspect., 114(1): 124–129.
Wasserman, G.A., Liu, X., Parvez, F., Factor-Litvak, P., Ahsan, H., Levy, D., Kline, J., van Geen, A., Mey, J., Slavkovich, V., Siddique, A.B., Islam, T. and Graziano, J.H. (2011). Arsenic and manganese exposure and children's intellectual function. Neurotoxicology, 32(4): 450–457.
Weber, S., Dorman, D.C., Lash, L.H., Erikson, K., Vrana, K.E. and Aschner, M. (2002). Effects of manganese (Mn) on the developing rat brain: Oxidative-stress related endpoints. Neurotoxicology, 23(2): 169–175.
WHO (2004). Concise international chemical assessment document 63: Manganese and its compounds: Environmental aspects. World Health Organization, ISBN 92 4 153063 4.
WHO (2007). Standard for infant formula and formulas for special medical purposes intended for infants. CODEX STAN 72-1981. World Health Organization (WHO) and Food and Agriculture Organization of the United Nations (FAO) (ed.). Codex Alimentarius (originally published in 1981 and revised in 2007). Available at: www.codexalimentarius.org.
WHO (2011). Manganese in drinking water. Background document for development of WHO Guidelines for Drinking-water Quality. World Health Organization. Available at: www.who.int/water_sanitation_health/dwq/chemicals/manganese.pdf
Wong, P.K. (1988). Mutagenicity of heavy metals. Bull. Environ. Contam. Toxicol., 40: 597–603.
Woolf, A., Wright, R., Amarasiriwardena, C. and Bellinger, D. (2002). A child with chronic manganese exposure from drinking water. Environ. Health Perspect., 110(6): 613–616.
Wright, R.O. and Baccarelli, A. (2007). Metals and neurotoxicology. J. Nutr. 137(12): 2809–2813. Review.
Wright, R.O., Amarasiriwardena, C., Woolf, A.D., Jim, R. and Bellinger, D.C. (2006). Neuropsychological correlates of hair arsenic, manganese, and cadmium levels in school-age children residing near a hazardous waste site. Neurotoxicology, 27(2): 210–216.
Yokel, R.A., Lasley, S.M. and Dorman, D.C. (2006). The speciation of metals in mammals influences their toxicokinetics and toxicodynamics and therefore human health risk assessment. J. Toxicol. Environ. Health B, 9(1): 63–85.
Yoon, J.H. and Ahn, Y.S. (2015). A large, nationwide, longitudinal study of central nervous system diseases among Korean workers exposed to manganese. Parkinsonism Relat. Disord., 21(3): 194–198.
Yoon, M., Nong, A., Clewell, H.J., 3rd, Taylor, M.D., Dorman, D.C. and Andersen, M.E. (2009a). Lactational transfer of manganese in rats: predicting manganese tissue concentration in the dam and pups from inhalation exposure with a pharmacokinetic model. Toxicol. Sci., 112(1): 23–43.
Yoon, M., Nong, A., Clewell, H.J., 3rd, Taylor, M.D., Dorman, D.C. and Andersen, M.E. (2009b). Evaluating placental transfer and tissue concentrations of manganese in the pregnant rat and fetuses after inhalation exposures with a PBPK model. Toxicol. Sci., 112(1): 44–58.
Yoon, M., Schroeter, J.D., Nong, A., Taylor, M.D., Dorman, D.C., Andersen, M.E., et al. (2011). Physiologically based pharmacokinetic modeling of fetal and neonatal manganese exposure in humans: describing manganese homeostasis during development. Toxicol. Sci., 122(2): 297–316.
Yu, X., Cao, L. and Yu, X. (2013). Elevated cord serum manganese level is associated with a neonatal high ponderal index. Environ. Res., 121(0): 79–83.
Yu, X., Zhang, J., Yan, C. and Shen, X. (2014). Prenatal exposure to manganese at environment relevant level and neonatal neurobehavioral development. Environ. Res., 133(0): 232–238.
Yukon Environmental Health Services (2014). Personal communication with P. Brooks.
Zacheus, O.M., Lehtola, M.J., Korhonen, L.K., Martikainen, P.J. (2001). Soft deposits, the key site for microbial growth in drinking water distribution networks, Water Res., 35(7): 1751–1765.
Zhao, F., Cai, T., Liu, M., Zheng, G., Luo, W. and Chen, J. (2009). Manganese induces dopaminergic neurodegeneration via microglial activation in a rat model of manganism. Toxicol. Sci., 107(1): 156–64.
Zheng, W., Kim, H. and Zhao, Q. (2000). Comparative toxicokinetics of manganese chloride and methylcyclopentadienyl manganese tricarbonyl (MMT) in Sprague-Dawley rats. Toxicol. Sci., 54: 295–301.
Zheng, W., Ren, S. and Graziano, J.H. (1998). Manganese inhibits mitochondrial aconitase: A mechanism of manganese neurotoxicity. Brain Res., 799: 334–342. [As cited in Health Canada, 2010]
Zlotkin, S.H., Atkinson, S. and Lockitch, G. (1995). Trace elements in nutrition for premature infants. Clin. Perinatol., 22(1): 223–240. [As cited in Health Canada, 2010]
Zota, A.R., Ettinger, A.S., Bouchard, M., Amarasiriwardena, C.J., Schwartz, J., Hu, H. and Wright, R.O. (2009). Maternal blood manganese levels and infant birth weight. Epidemiology, 20(3): 367–373.
Zwingmann, C., Leibfritz, D. and Hazell, A.S. (2003). Energy metabolism in astrocytes and neurons treated with manganese: relation among cell-specific energy failure, glucose metabolism, and intercellular trafficking using multinuclear NMR-spectroscopic analysis. J. Cereb. Blood Flow Metab., 23: 756–771.
Appendix A: List of Acronyms
- AI
- adequate intake
- ANSI
- American National Standards Institute
- AO
- aesthetic objective
- ATP
- adenosine triphosphate
- BBB
- blood-brain barrier
- CAS
- Chemical Abstracts Service
- CI
- confidence interval
- CNS
- central nervous system
- CSF
- cerebral spinal fluid
- DBP
- disinfectant by-product
- DO
- dissolved oxygen
- DOC
- dissolved organic carbon
- EPA
- Environmental Protection Agency (U.S.)
- GAC
- granular activated carbon
- GCI
- McCarthy general cognitive index
- HAAs
- haloacetic acids
- HBV
- health-based value
- ICP-AES
- inductively coupled plasma–atomic emission spectroscopy
- ICP-MS
- inductively coupled plasma–mass spectrometry
- IQ
- intelligence quotient
- LOAEL
- lowest-observed-adverse-effectlevel
- MAC
- maximum acceptable concentration
- MDL
- method detection limit
- MnB
- manganese concentration in blood
- MnH
- manganese concentration in hair
- MnUmbi
- manganese concentration in umbilical serum
- MnW
- manganese concentration in water
- MOB
- manganese-oxidizing bacteria
- MRL
- minimum reporting level
- MIREC
- Maternal-Infant Research on Environmental Chemicals
- NOAEL
- no-observed-adverse-effect level
- NBNA
- neonatal behavioural neurological assessments
- NOM
- natural organic matter
- NSF
- NSF International
- ORP
- oxidation/reduction potential
- PBPK
- physiologically based pharmacokinetic
- POE
- point-of-entry
- POU
- point-of-use
- PQL
- practical quantitation level
- PVC
- polyvinyl chloride
- RO
- reverse osmosis
- SCC
- Standards Council of Canada
- SD
- standard deviation
- SM
- Standard Method
- TDI
- tolerable daily intake
- TDS
- total diet study
- Tf
- transferrin
- THMs
- trihalomethanes
- UCMR3
- third Unregulated Contaminant Monitoring Rule
- UL
- tolerable upper intake level
- WHO
- World Health Organization
Appendix B: Provincial and territorial anticipated impacts
Please note that this information is not available in both official languages because the source of the information is not subject to the Official Languages Act.
Prince Edward Island
Manganese in concentrations in Prince Edward Island drinking water are generally low, and the proposed guidelines would have a relatively small impact in the Province. It is not expected that any municipal water supply system will need to take action to meet the proposed health based guideline or aesthetic objective. The health based guideline of 0.1 mg/L is expected to have a minimal impact on private well owners, as it is likely they would be taking action to reduce manganese levels at this concentration on an aesthetic basis in any case. Assuming that acceptance by homeowners of elevated manganese concentrations is based on actual adverse effects rather than guideline levels, the potential effect on private well owners may be primarily in relation to the revised aesthetic objective and it's influence on real estate transactions, with a slight increase (up from ~3% to ~5%) in private wells exceeding the proposed aesthetic guideline.
Newfoundland and Labrador
The Province of Newfoundland and Labrador is responsible for extensive monitoring for inorganic parameters including manganese in the province. Manganese monitoring is conducted semi-annually for all public water supplies in the province, and quarterly for populations larger than 5,000.
Of the 305 surface water sources in the province, 258 (85%) are protected under the Water Resources Act. Of the 198 groundwater sources in the province, 59 (30%) are protected under the Water Resources Act. This provides for an extensive source water protection program that reduces the risk of contamination for drinking water sources. A total of 76 public drinking water supplies (both surface water and groundwater) will exceed the proposed MAC of 0.1 mg/L.
A total of 232 public drinking water supplies (both surface water and groundwater) may exceed the proposed AO of 0.02 mg/L periodically. Of these, 92 may be systems that have never had manganese exceedances in the past. In the 2013-14 fiscal year, there were 102 manganese exceedances in the province. Additionally, an unknown number of private wells may exceed the proposed guidelines.
The majority of Newfoundland and Labrador’s drinking water systems do not have any treatment other than disinfection as 68% of the systems service very small populations (less than 500 people). Due to this fact, the cost of implementing this guideline to remove manganese from systems where exceedances are expected will be extremely expensive, especially as most of the systems that will require treatment are located in very small communities that lack resources.
Treatment for manganese is currently used in only 6 distribution systems in the province with marginal effectiveness.
The province will continue to monitor for manganese at the current frequency, and to encourage source water protection for all public water supplies. It will also introduce manganese/iron removal treatment systems as necessary for public water supplies at risk of exceeding the guidelines.
Nova Scotia
The revised guideline proposes a maximum acceptable concentration (MAC) for manganese of 100 µg/L and a reduction to the current aesthetic objective (AO) from 50 µg/L to 20 µg/L. The proposed development of a health-based guideline for manganese and a reduction to the current aesthetic objective will likely result in moderate to significant impact for Nova Scotia.
Nova Scotia's drinking water program consists of public and private supplies. Public drinking water supplies include municipal (approved) and registered facilities. 64.7% (613,784 persons) of the population are serviced by a municipal system; 1.3% (12,200 persons) are serviced by a registered facility; and the remaining 34% (322,754 persons) rely on the use of a private drinking water supply.
Nova Scotia Environment (NSE) regulates water quality at municipal and registered public drinking water facilities. There are currently 1545 registered facilities. As part of our evaluation, NSE analyzed available data from 53% of these facilities. The data identified that 43% exceed an AO of 20 µg/L with 22% exceeding a MAC of 100 µg/L. Existing data included as part of our evaluation for registered facilities prevents an accurate determination of true impact as treatment information maintained by NSE is not current and we do not have data for all of our registered facilities. NSE regulates 83 municipal drinking water facilities. Recent data identifies three facilities exceeding a MAC of 100 µg/L for manganese with 14 facilities exceeding an AO of 20 µg/L in treated water.
NSE does not regulate water quality at private residences; however, we wanted to include them in our impact analysis as 34% of our population relies on the use of a private drinking water supply. Analysis of available data from various sources coupled with knowledge of local geology and unserviced areas suggests that up to 70,000 private drinking water supplies may be impacted. As NSE does not maintain water quality data for private drinking water supplies, it is not possible to provide a determination of true impact with any certainty.
For Nova Scotia, the proposed establishment of a MAC for manganese will require: the development of a communication strategy to encourage homeowners on private supplies to have their water tested for manganese; the development of risk communication messages in consultation with our Medical Officers of Health ( MOH); the development of educational materials; and the development of a compliance/ enforcement strategy for our public systems.
The proposed revisions will likely result in challenges for our public drinking water supplies, particularly registered facilities; homeowners relying on the use of private drinking water supplies; and the Compliance Division of NSE. Specific challenges are likely to include: increased rates of non-compliance as an estimated 22 % of registered facilities will be out of compliance with the new health-based guideline; increased staff resources to address increased rates of non-compliance; increased sampling costs; and costs associated with the purchase, installation, operation and maintenance of treatment devices.
New Brunswick
New Brunswick is supportive of minimizing exposure of manganese from drinking water, now that it has been determined that a health based value is necessary to be protective.
The impact to New Brunswick may be substantial. Manganese is a very common element in New Brunswick drinking water. When reviewing drinking water quality results, we can estimate approximately 1/3 of regulated municipal raw water sources may not be compliant with the new MAC. Proper source water characterization will likely have to be undertaken for many regulated systems. Some systems already have manganese treatment in place to address aesthetic concerns, but for those that don’t, there may be a need to construct new water treatment plants. Associated costs could be significant. Improvements to water distribution systems may be required. Those municipal systems with existing water treatment plants may need to be optimized and additional post-treatment monitoring will be required as well as additional distribution system monitoring.
There are numerous Crown Systems with historical values that exceeded the new MAC. Further investigation here will be needed to determine next steps.
Approximately 30% of all private wells in NB will not be compliant with the new MAC. A rough estimate implicates approximately 30,000 wells.
Additional discussion will be required with stakeholders to discuss how to proceed with implementation of the proposed Guideline and how to manage monitoring and source-water characterization.
Quebec
Au Québec, étant donné que le manganèse ne fait pas l'objet d'une norme au Règlement sur la qualité de l'eau potable, les résultats de manganèse disponibles découlent des campagnes d'échantillonnage réalisées par le ministère du Développement durable, de l’Environnement et de la Lutte contre les changements climatiques dans le cadre du Programme de surveillance de la qualité de l’eau potable. Ainsi, de novembre 2010 à mars 2014, 410 analyses du manganèse ont été réalisées dans 107 d’installations de production d’eau potable alimentées en eau souterraine.
Parmi ces résultats d’analyse, 31 (7,6 %) ont montré un résultat supérieur à la CMA proposée de 0,1 mg/L (100 μg/L), ce qui concerne 18 (16,8 %) des installations de production d’eau potable ayant fait l’objet d’une analyse. Par ailleurs, 52 (12,7 %) résultats de manganèse dépassant l’objectif esthétique proposé de 0,02 mg/L (20 μg/L) ont été observés pour 29 (27 %) des installations visitées. Considérant les résultats d'analyse disponibles et le très grand nombre d’installations de production d’eau potable qui s’approvisionnent en eau souterraine au Québec, les impacts attendus de l’ajout d’une norme pour le manganèse à la réglementation, en fonction de la révision de la recommandation publiée par Santé Canada, seraient relativement importants.
Ontario
The proposed guideline value will have minimal impact in Ontario. Ontario currently has an aesthetic objective for manganese that is lower than the proposed guideline.
The aesthetic objective being proposed in the consultation is lower than the current aesthetic objective and may impact small drinking water systems.
Manitoba
A review of the available manganese data shows that the proposed change to the aesthetic objective (from 0.05 to 0.02 mg/L) and, more importantly, establishment of a health-based guideline of 0.1 mg/L would have a significant impact on Manitoba’s public and semi-public water systems. Approximately 30% of public water systems and 20% of semi-public water systems licenced to date show manganese levels at the treatment plant at or above 0.02 mg/L. About 10% of public water systems and a similar proportion of licenced semi-public water systems show manganese levels at the proposed 0.1 mg/L MAC or above. Data from the water treatment plant does not take into consideration potential ‘legacy manganese’ that may have accumulated within distribution lines or consumers’ service lines over the years as part of sediment or pipe scale.
Saskatchewan
The Water Security Agency (WSA) has reviewed the proposed guideline technical document for manganese in drinking water and agrees with the proposed MAC of 100 µg/L and an aesthetic objective of 20 µg/L for total manganese in drinking water. Drinking water analytical results for 3643 samples collected over the last 5 years (2010 to present) from the distribution systems of municipal waterworks regulated by the WSA showed that total manganese levels exceeded the proposed MAC in 108 sampling locations or distribution systems of communities in the province.
Nearly 50% of the ground water treatment systems in Saskatchewan contain significant amounts of iron and manganese in their raw water, and most of these systems have manganese greensand filters to remove manganese from their treated water. These systems adopt a combination of processes (e.g., oxidation using potassium permanganate followed by adsorption/and or filtration in manganese greensand filters) to remove manganese from drinking water. The affected communities continue to monitor manganese levels in treated water as per monitoring requirements specified in the provincial document “Municipal Drinking Water Quality Monitoring Guidelines - EPB 202” and in accordance with operational permit conditions. The proposed MAC for manganese may pose a significant compliance challenge for affected communities as WSA will be compelled to require upgrading once revised standards are adopted in the province. The Water Security Agency will work closely with the affected communities to ensure compliance in the future. Cost estimation for treatment upgrade for these affected communities is not possible at this time.
Alberta
In Alberta it is estimated that around 3% of municipal drinking-water systems will not meet the proposed MAC with a further 15% not being able to meet the proposed revised AO. No estimate of costs are available at this time as the range of potential solutions will be unique to each individual system and may range from changes in operational practice (such as blending for groundwater systems) through to capital expenditure to provide additional process capacity.
British Columbia
It is anticipated that the proposed change to the manganese guidelines to make the aesthetic objective (AO) more stringent as well as the addition of a maximum acceptable concentration (MAC) will have significant impact on many British Columbia water supply systems.
The change in AO from 0.05 mg/L to 0.02 mg/L would double the number of systems exceeding the objective (200-400 in IHA alone), however this is a minor concern as there is no health impact associated with this change. As manganese concentrations at levels near the AO cause unsightly water, this is often motivation to address this issue even though there is no health concern.
More significant is the proposed addition of a maximum acceptable concentration (0.1 mg/L) based on studies citing an association between exposure to manganese in drinking water and neurological effects in children. This will mean significantly different messaging from what health officials have historically given regarding manganese. It will also require the need for clear and meaningful advice to those that have been consuming water sources that exceed the proposed MAC, who have previously been told that elevated manganese is merely an aesthetic concern.
It is estimated that well over 400 existing water supply systems in BC would exceed the proposed MAC, most of which would be smaller sized systems. Health Canada asserts that the new MAC and AO for manganese should be achievable for most treatment plants. Water suppliers with manganese concentrations consistently above the MAC will arguably need to plan, finance and install such treatment. This will particularly challenge small water systems, as a high proportion of these are on groundwater, and they have limited resources to pay for installation and operation of treatment.
The proposed MAC may also limit options for new sources of water when expanding systems in high manganese regions of the province. Given that manganese is more prevalent in groundwater than surface water, the new proposed manganese guidelines may further pose a challenge for private well owners in BC, which are not captured in the numbers reported above. In summary, these would have significant resource implications on health authorities, water suppliers and ultimately users.
The overall financial, social and health impacts of adopting this guideline are difficult to quantify, however it is reasonable to anticipate that for communities with elevated manganese in drinking water, short term costs of treatment will be balanced against long term diminished potential lifetime earnings as well as long term cost of increased social services and education associated with potential neurological effects.
Yukon
It is not anticipated that the guideline technical document for manganese will have a significant impact on Yukon large public drinking water systems.
Northwest Territories
No impact paragraph has been provided by the territory.
Nunavut
No impact paragraph has been provided by the territory.
Footnotes
Page details
- Date modified: