Proposed Residential Indoor Air Quality Guidelines for Benzene
Preamble
Health Canada assesses the health risks posed by specific indoor pollutants in residential environments and provides recommendations on how to reduce those risks. Residential Indoor Air Quality Guidelines (RIAQG) summarize the known health effects, pollutant sources, and exposure levels in Canadian homes and characterize the risks to health, based on the best scientific data available. Proposed exposure limits (also referred to as guideline values) for short- and/or long-term exposure to the pollutant are developed, representing indoor air concentrations below which health effects are unlikely to occur. The proposed guideline values take into account the reference concentrations (RfC) and/or risk-specific concentrations (RSC) for the pollutant and the feasibility of achieving such levels through control of indoor sources. The RIAQG also include recommendations for controlling sources or other actions to reduce exposure to the pollutant.
The RIAQG and guidance documents serve as a scientific basis for activities to evaluate and reduce the risk from indoor air pollutants, including:
- assessments by public health officials of health risks from indoor air pollutants in residential or similar environments;
- performance standards that may be applied to pollutant-emitting materials, products, and devices, so that their normal use does not lead to air concentrations of pollutants exceeding the proposed guideline values; and
- communication products informing Canadians of actions they can take to reduce their exposure to indoor air pollutants and to help protect their health.
The guideline values proposed in RIAQG are intended for professional audiences to support evaluation of potential risk and provide justification for risk management actions. In the majority of cases, Health Canada does not recommend that individuals measure concentrations of indoor air pollutants in the home, but instead focus on implementation of risk mitigation strategies to improve indoor air quality.
The RIAQG and guidance documents replace a series of exposure limit values for indoor air pollutants taken from a report entitled Exposure Guidelines for Residential Indoor Air Quality (Health Canada 1987).In addition to updates for the substances included in the 1987 report, guidelines or guidance documents will be developed for other substances that are identified as having the potential to affect human health in the indoor environment.
This document updates and replaces the previous Guidance for Benzene in Residential Indoor Air: Science Assessment Document (Health Canada 2013a).
Table of contents
- List of tables
- List of figures
- Executive summary
- Introduction
- 1.0 Physical and chemical characteristics
- 2.0 Concentrations in indoor and outdoor air
- 3.0 Sources in the air
- 4.0 Toxicokinetics
- 5.0 Health effects
- 6.0 Derivation of reference concentrations
- 7.0 Proposed guidelines
- 8.0 References
- Appendix A: List of acronyms and abbreviations
- Appendix B: Human exposure studies
- Appendix C: Toxicological studies
- Appendix D: Other inhalation reference exposure levels
List of tables
- Table 1. Physical and chemical properties of benzene
- Table 2. Indoor, outdoor, and personal exposure concentrations (μg/m3) of benzene measured in Canada
- Table 3. Relative change in mean indoor benzene levels by predictor in Canadian studies
- Table 4. Proposed guideline value for benzene for indoor environments
- Table B1. Short-term exposure studies in humans
- Table B2. Long-term exposure studies in humans: Respiratory toxicity
- Table B3. Long-term exposure studies in humans: Reproductive and developmental toxicity
- Table B4. Long-term exposure studies in humans: Hematotoxicity and immunotoxicity
- Table B5. Long-term exposure studies in humans: Other effects
- Table B6. Genotoxicity studies in humans
- Table B7. Carcinogenicity
- Table C1. Short-term exposure studies in experimental animals
- Table C2. Genotoxicity studies in experimental animals
- Table D1. Other short-term inhalation reference exposure levels
- Table D2. Other inhalation reference exposure levels for long-term non-cancer effects
- Table D3. Other inhalation unit risks for carcinogenic effects
List of figures
- Figure 1. Distribution of benzene concentrations in indoor air by season across studies conducted by Health Canada
- Figure 2. Distribution of I/O ratios by season across studies conducted by Health Canada
- Figure 3. Proposed pathway for the metabolism of benzene
Executive summary
Guideline value | Concentration | Critical effect(s) | |
---|---|---|---|
μg/m3 | ppb | ||
Long-term | 0.6 | 0.2 | Leukemia |
The proposed long-term guideline value for benzene is 0.6 μg/m3 (0.2 ppb). A short-term guideline value was not derived for indoor air exposure.
Levels of benzene in many homes in Canada may exceed the guideline value and may pose a health risk. It is therefore recommended that homeowners and residents reduce exposure to benzene by ensuring adequate ventilation and controlling or eliminating indoor sources.
Background
Benzene is a volatile organic compound with a high vapour pressure that quickly evaporates into the air when released into the environment. Indoor concentrations of benzene are generally higher than outdoor concentrations.
Benzene is included on Schedule 1 of the Canadian Environmental Protection Act. In 1993, Health Canada concluded that benzene may constitute a danger to human life or health in Canada as a non-threshold carcinogen. The concentration that corresponds to a 5% increase in mortality due to acute myelogenous leukemia was calculated to be 14.7 × 103 μg/m3, and the corresponding exposure/potency index for benzene in Canada, 3.0 × 10-4. In 2013, Health Canada published the Guidance for Benzene in Residential Indoor Air, which made the qualitative recommendation that individuals take action to reduce exposure to benzene indoors as much as possible, due to a low but non-negligible risk of cancer at indoor exposure concentrations. In 2021, Health Canada recommended the use of a toxicological reference value of 1.6 × 10 -2 (mg/m3) -1 unit risk for risk assessments of inhalation exposure to benzene at federal contaminated sites, based in part on the 2013 Guidance (Health Canada 2021b).
In 2019, the Health Canada Indoor Air Program identified benzene as a priority for an updated human health risk assessment and for development of Residential Indoor Air Quality Guidelines based on several considerations. These include the identification of new health effects data at environmental (non-occupational) exposure concentrations, and completion of the Residential Attached Garage Intervention study, which examined risk mitigation approaches for benzene infiltration from attached garages.
This Guideline document reviews the exposure, toxicological and epidemiological research on benzene published since the previous Health Canada Guidance, as well as the conclusions from comprehensive reviews conducted by internationally recognized health and environmental organizations. This document proposes a long-term indoor air guideline value for benzene, which is intended to minimize risks to human health and to support the development of actions to limit benzene emissions indoors. This document also recommends various risk mitigation measures to reduce exposure to benzene in indoor air.
Exposure and sources
Median residential indoor benzene levels measured by Health Canada in multiple cities and two First Nations communities in Canada from 2005 to 2019 ranged from 0.4 to 2.2 μg/m3, and the 95th percentiles ranged from 2.4 to 18.6 μg/m3. Preliminary data from a Health Canada study indicate that benzene levels in newly built homes also fall within these ranges. In 2019, the national average concentration of outdoor benzene at National Air Pollution Surveillance program stations was 0.5 μg/m3.
Benzene is a natural component of petroleum products such as gasoline and crude oil and is added to unleaded gasoline in small quantities to enhance performance. It is also produced by combustion and other anthropogenic sources. Seven hundred thousand tonnes of benzene were produced in Canada in 2019. The primary industrial use of benzene is the production of organic chemicals that are used to manufacture products such as plastics, resin, nylon, synthetic fibres, lubricants, rubbers, dyes, detergents, and pesticides.
Key sources of ambient (outdoor) benzene concentrations are vehicle emissions and industrial emissions. Other major outdoor sources of benzene include releases from gas stations during normal operations and releases from tanker truck fuel delivery, wildfires, and residential wood burning.
Indoor benzene concentrations in Canadian homes are 1.3–7.9 times higher than outdoor concentrations, with the highest ratios of indoor-to-outdoor concentration observed in homes with people who smoke. The presence of an attached garage, along with the storage of gasoline, paints, and solvents in the garage and home, was associated with higher levels of benzene in the home. Indoor smoking and increased outdoor benzene concentrations are also associated with higher indoor levels of benzene. Increased ventilation—for example, by increasing the air exchange rate and opening windows more often—use of an exhaust fan in the garage, and proper sealing of the garage/home interface have been shown to reduce indoor benzene concentrations..
Health effects
Health effects are associated with benzene exposure at both occupational (very high, for example, >3.2 mg/m3 or 1 ppm) and environmental (normally very low, for example, <10 μg/m3 or 3 ppb) concentrations. At very high exposures, such as in the levels observed in occupational settings and in environmental releases, benzene has been shown to cause central nervous system (CNS) and neurological symptoms, respiratory symptoms, and hematotoxic effects.
Epidemiological studies of benzene exposure in indoor residential settings report impaired lung function, increased asthma prevalence, and asthma symptoms in adults and children. Personal and ambient exposure to benzene during pregnancy has been associated with preterm birth and developmental effects such as reduced head circumference and low birth weight. Other non-cancer effects in humans include neurological effects on hearing and cognition, as well as effects on glucose metabolism. Due to study design, it is not clear to what extent these effects may be due to the presence of co-pollutants.
Hematotoxicity is a well-established non-cancer effect of benzene exposure that has been reported to occur in occupational settings. Effects at occupational exposure levels include decreased blood cell counts and effects on precursor cell populations in bone marrow. Altered blood cell counts have also been reported in biomonitoring studies of the general population; however, some effects are inconsistent with those reported at higher occupational exposures.
Benzene is genotoxic and is classified as a human carcinogen by Health Canada (Environment Canada and Health and Welfare Canada 1993), the United States Environmental Protection Agency (US EPA 2002), and the International Agency for Research on Cancer (IARC 1987, 2018). IARC concluded that benzene causes acute myeloid leukemia and acute non-lymphocytic leukemia in adults, and that the data support a positive association between benzene exposure and leukemia in children. Previous cancer risk assessments were based on very high exposure levels in occupational settings; however, there is some uncertainty about the suitability of extrapolating health effects from high occupational exposure to low residential concentrations. A linear extrapolation based on key occupational cohorts indicates that there is a low but non-negligible risk of cancer in many Canadian homes.
Several populations may be susceptible or more highly exposed to benzene. Biomonitoring studies in Canada report higher levels of blood benzene or urinary metabolites in people who smoke, in South Asian Canadians, and in Indigenous women. Women are expected to be more susceptible to health effects related to benzene exposure than men in the same setting due to metabolic differences. Children are also expected to be more susceptible due to physiological and metabolic differences compared to adults. Genetic polymorphisms that affect benzene metabolism and increase susceptibility to adverse effects may be present in a significant proportion of the population.
Proposed long-term residential indoor air quality guideline value
Hematotoxicity (reduced white blood cells) is the most sensitive non-cancer endpoint for long-term benzene exposure. The lower bound of the benchmark concentration (BMCL) for hematotoxicity in an occupational study of cumulative exposure to benzene is 26 μg/m3 (8.1 ppb).
To derive a reference concentration (RfC), an uncertainty factor of 30 was applied to the hematotoxicity BMCL to account for the use of healthy workers as subjects (10-fold) and to account for database uncertainty regarding physiologically based pharmacokinetic modelling at environmentally relevant exposures (3-fold). The proposed RfC for this endpoint is 0.9 μg/m3 (0.3 ppb).
The previous Health Canada Guidance concluded that, based on key cancer risk assessments, the most reasonable inhalation unit risk range was 2.2 × 10-6 to 1.6 × 10 -5 (μg/m3)-1.This range corresponds to concentrations of 0.6–4.5 μg/m3 associated with risk of 1 × 10-5. More recent cancer risk assessments also fall into this range, indicating that there is no need to revise the previous conclusion at this time.
The proposed risk-specific concentration (RSC) for cancer, 0.6 μg/m3 (0.2 ppb), corresponds to an inhalation unit risk of 1.6 × 10 -5 (μg/m3) -1 and an acceptable cancer risk of 10-5. Hematotoxicity has been proposed as a potential precursor event for benzene-related carcinogenicity. However, the mode of action for benzene toxicity is still not yet fully understood and the RSC for leukemia is a more health protective value than the RfC for hematotoxicity. Therefore, the proposed long-term RSC for benzene toxicity is 0.6 μg/m3 (0.2 ppb).
In order to determine the proposed guideline values, the long-term RfC or RSC is first compared to available exposure data from Canadian homes to evaluate the feasibility of achieving the RfC or RSC through the control of indoor sources. If the RfC or RSC is considered feasible, the identified value is set as the proposed guideline value. If not, a higher concentration may be selected, while still targeting a guideline value that is protective of health in consideration of current evidence.
In the present assessment, the criteria guiding the determination of the value for both the proposed short- and long-term guideline values for benzene are:
- a value that is potentially achievable in Canadian homes in the absence of significant sources of indoor benzene; and
- a value that is not associated with appreciable health effects, considering the derived reference exposure levels and currently available evidence.
Health Canada data indicate that the long-term RSC falls within the range of median indoor air concentrations measured in homes in Canada. The RSC was derived using the most recent and relevant scientific information. Additionally, data from Health Canada and other studies indicate that benzene levels could be significantly reduced in homes through ventilation and source control. As the average outdoor air concentration of benzene in Canada is lower than the RSC, ventilation, along with source control, can be considered an effective strategy to reduce indoor benzene concentrations. Therefore, the proposed long-term guideline value for benzene is 0.6 μg/m3 (0.2 ppb).
When a measured benzene concentration is compared to the long-term guideline value, the sampling time that is used should be at least 24 hours. Averaging the results of repeated samples, taken in different seasons, will provide a more representative estimate of long-term exposure for comparison to the long-term guideline value.
Long-term or chronic non-cancer inhalation reference exposure levels for benzene have been derived by the US EPA (30 μg/m3, 2002), the Agency for Toxic Substances and Disease Registry (10 μg/m3, 2007), l'Agence Nationale de Sécurité Sanitaire de l'Alimentation, l'Environnement et du Travail (ANSES) (10 μg/m3, 2008), the California Office of Environmental Health Hazard Assessment (3 μg/m3, 2014), and the Texas Commission on Environmental Quality (TCEQ) (84 μg/m3, 2015). Each of these values was derived based on a key health effect of hematotoxicity, prior to the publication of the key study for the non-cancer RfC derived in this assessment.
Inhalation unit risk values for cancer have been reported by the US EPA (2.2 × 10-6 to 7.8 × 10-6[μg/m3]-1), World Health Organization (WHO) (6 × 10-6 [μg/m3]-1), TCEQ (2.2 × 10-6[μg/m3]-1), ANSES (6 × 10-6 [μg/m3]-1), National Institute for Public Health and the Environment of the Netherlands (RIVM) (5 × 10-6 [μg/m3]-1) and the German Environment Agency (Umweltbundesamt) (1 × 10-5[μg/m3]-1). These values correspond to air concentrations of 1.3 to 4.5 μg/m3 (US EPA), 1.7 μg/m3 (WHO), 4.5 μg/m3 (TCEQ), 0.6 μg/m3 (Health Canada Contaminated Sites Division), 2 μg/m3 (ANSES), 2 μg/m3 (RIVM) and 1 μg/m3 (German Environment Agency)at an acceptable cancer risk level of 1 × 10-5.
Risk management recommendations
Exposure to benzene in indoor air should be limited by ensuring adequate ventilation and controlling or eliminating indoor sources using the strategies described below. Many of these measures will also help lower the concentrations of other indoor air contaminants, leading to a general improvement in indoor air quality.
- If you have an attached garage:
- Consider installing and using a garage exhaust fan;
- Ensure that the interface between the attached garage and the home is properly sealed; and
- Avoid idling your car, snow blower, lawnmower, or any gas-powered equipment in the garage.
- Avoid storing gasoline, paint, solvents or other chemicals in the home or attached garage. These products should be stored in tightly sealed containers, and, if possible, kept in a separate building that isn't connected to your home's ventilation system.
- Switch from gasoline-powered home equipment like lawn mowers, leaf blowers and snow blowers to battery-powered equipment.
- Do not smoke indoors.
- Increase ventilation in the home:
- By using natural ventilation strategies like opening windows and interior doors, if possible (check the outdoor air quality conditions in your region before opening windows);
- By using mechanical ventilation strategies like using fans with outside venting, forced air ventilation, energy recovery ventilators (ERVs) and heat recovery ventilators (HRVs); and
- Pay attention to signs that the ventilation rate is too high, such as dry air and drafts.
- For more information, refer to the factsheet: Ventilation and the Indoor Environment and the infographic: Ventilation and Indoor Air Quality.
- When outdoor air quality is poor (for example, due to
high levels of traffic or wildfire smoke events):
- Keep windows and doors closed, and use air conditioning to maintain a comfortable temperature indoors;
- Properly seal windows and doors with weather stripping; and
- If possible, set your ventilation system to recirculate and bring in fresh air when outdoor air has improved.
- For more information, refer to the infographic: Protecting your Indoor Air from Outdoor Pollutants.
- Ensure that fireplaces and wood stoves are properly
installed and maintained, and vent pollutants to the outdoors.
- For more information, refer to Wood Smoke and Indoor Air.
Introduction
This document is an update of the previous Guidance for Benzene in Residential Indoor Air: Science Assessment Document (Health Canada 2013a) and addresses the available scientific literature on health effects and Canadian exposure to benzene published in the intervening years. A search was conducted of the scientific literature on health effects of and exposure to benzene published between January 2009 and June 2020. Key studies from this search are reviewed herein, along with the conclusions of comprehensive reviews conducted by internationally recognized health and environmental organizations.
1.0 Physical and chemical characteristics
Benzene is a clear, colourless or pale-yellow volatile liquid with an aromatic odour at room temperature. It is highly flammable and has a relatively low boiling point (80.1°C). It has a high vapour pressure (10.1 kPa–13.2 kPa at 25°C) and quickly evaporates into the air. Benzene is soluble in water and miscible with most organic solvents. The physical and chemical properties of benzene are summarized in Table 1 (Health Canada 2013a, ATSDR 2007).
Property | Value | Chemical structure |
---|---|---|
CAS registry number | 71-43-2 | |
Molecular formula | C6H 6 | |
Molecular weight | 78.11 g/mol | |
Density | 0.878 g/cm3 at 25°C | |
Vapour pressure | 10.1 kPa–13.2 kPa at 25°C | |
Water Solubility | w/w: 0.188% at 25°C | |
Boiling point | 80.1°C | |
Flash point | -11°C (closed cup) | |
Octanol/water partition coefficient (logKow) | 2.13 | |
Common synonyms | Annulene, benzole, coal naphtha, cyclohexatriene, petrobenzene, phenyl hydride, pyrobenzol, pyrobenzole | |
Conversion factors | 1 ppm = 3.2 mg/m3 at 25°C and 1 atm (760 mm Hg) 1 mg/m3 = 0.31 ppm |
2.0 Concentrations in indoor and outdoor air
2.1 Outdoor concentrations
The National Air Pollution Surveillance program indicated that in 2019, across 72 monitoring stations, the annual national average concentration of ambient benzene was 0.50 μg/m3. This includes a rural average of 0.21 μg/m3 (12 stations) and an urban average of 0.40 μg/m3 (39 stations). The annual average concentration at urban transportation-influenced sites was 0.64 μg/m3, and 0.77 μg/m3 at locations with a major stationary emissions source (for example, industrial, or traffic-influenced sites) (Environment and Climate Change Canada 2021).
In Health Canada residential studies conducted in Edmonton, Regina, Halifax, Windsor, Ottawa, and Montreal, median outdoor concentrations of benzene ranged from 0.2 to 0.8 μg/m3. The 95th percentiles ranged from 0.6 to 2.7 μg/m3 (Health Canada 2010a, 2010b, 2012, 2013b, 2021a; Mallach et al. 2017; Goldberg et al. 2015; Weichenthal et al. 2013; Zhu et al. 2005). Data from these studies are shown in Table 2.
The mean outdoor benzene concentration in residential areas is 4.7 μg/m3 in Europe and 6.3 μg/m3 in the United States (US) (Sekar 2019). In 2013, mean benzene levels measured across 343 monitoring stations in the US ranged from 0 μg/m3 in a rural setting to 4.4 μg/m3 in an urban industrial location (IARC 2018). Mean outdoor air concentrations in France (measured in 2005) were 1 μg/m3 in semi-urban and rural sites, 1–3 μg/m3 in urban sites and 1.5–6.5 μg/m3 in high traffic areas (ANSES 2008). In 2014, the European Environment Agency reported no exceedances of the 5 μg/m3 outdoor benzene limit in 42 European countries (IARC 2018).
2.2 Indoor concentrations
In Health Canada studies using passivated canisters in Edmonton, Halifax, Regina, Windsor, Ottawa, and Montreal, median indoor concentrations of benzene ranged from 0.4 to 2.2 μg/m3; the 95th percentiles ranged from 2.4 to 18.6 μg/m3. Similar results were reported in a First Nations reserve in southern Manitoba. The highest levels were found in Ottawa, Ontario, in a home with attached garages. Personal monitoring was also conducted in Windsor: median concentrations of benzene ranged from 1.5 to 1.6 μg/m3 and the 95th percentiles ranged from 4.6 to 9.2 μg/m3 (Health Canada 2010a, 2010b, 2012, 2013b, 2021a; Mallach et al. 2017; Goldberg et al. 2015; Weichenthal et al. 2013; Zhu et al. 2005; Kovesi et al. 2022). Data from these studies are shown in Table 2.
Similar benzene concentrations were observed in other Canadian studies, including homes in Quebec City, Nunavik, and Ottawa, and in a First Nations community in Sioux Lookout Zone, Ontario, as well as in the Canadian Health Measures Survey (CHMS). These studies used different sampling and analysis methods and were therefore not included in the ranges given above (Health Canada 2021a; Li et al. 2019; Héroux et al. 2008; Zhu et al. 2005; National Research Council 2021).
In a recent Canadian study, indoor benzene concentrations were measured in the homes of 85 pregnant people, including Indigenous residents of local First Nations communities, living near unconventional natural gas extraction activities in northeastern British Columbia. The median and 95th percentile levels were 0.80 μg/m3 and 7.40μg/m3, respectively, which are similar to the indoor benzene levels reported in previous Canadian studies (Caron-Beaudoin et al. 2022).
Reported mean concentrations of indoor benzene ranged from 3.0 to 4.1μg/m3 in the US and from 1.6 to 15.3 μg/m3 in Europe (Sekar 2019). The median concentration of benzene in German multi-unit dwellings measured during the 2015–2017 period was 1.1μg/m3, with a 95th percentile of 4.5 μg/m3 (Umweltbundesamt 2020). In France, the median concentration in dwellings measured from 2003 to 2005 was 2.1μg/m3, with a maximum concentration of 22.8μg/m3 (ANSES 2008).
Location | Sampling period | Sampling methodFootnote a | Season | No. of homes | Smoking status | No. of samplesFootnote b | Median (μg/m3) | 95th%ile (μg/m3) | Study reference |
---|---|---|---|---|---|---|---|---|---|
INDOOR | |||||||||
Edmonton, Alberta | 2010 | Passivated canisters (7 days × 24 hours) |
Summer Winter |
50 50 |
NS | 328 337 |
0.6 1.2 |
4.1 4.0 |
Health Canada (2013b) |
Halifax, Nova Scotia | 2009 | Passivated canisters (7 days × 24 hours) | Summer Winter |
50 50 |
NS | 331 312 | 0.5 0.8 |
9.0 8.2 |
Health Canada (2012) |
Regina, Saskatchewan | 2007 | Passivated canisters (24 hours) | Summer Winter |
111 106 | NS S NS S |
91 13 84 21 |
0.9 2.2 1.1 1.8 |
13.7 13.3 4.7 6.8 |
Health Canada (2010a) |
Windsor, Ontario | 2005-2006 | Passivated canisters (5 days × 24 hours) |
Summer Winter |
46 47 |
NS | 428 456 |
1.4 1.3 |
14.1 4.9 |
Health Canada (2010b) |
Ottawa, Ontario | 2014 | Passivated canisters (48 hours) | Winter | 33Footnote c | NS | 62 (garage fan off) 61 (garage fan on) | 1.7 1.1 |
18.6 3.3 |
Mallach et al. 2017 |
Ottawa, Ontario (Newly built homes) | 2019-21 | Passivated canisters (96 hours) | All | 18 | — | 17Footnote d 13Footnote e | 1.1 0.4 |
5.9 3.6 |
Personal communication, Health Canada 2021a |
Ottawa, Ontario (Schools) | 2013 | Passivated canisters (6.5 hours) | Fall | 4 | — | 128 | 0.5 | 2.4 | MacNeill et al. 2016 |
Montreal, Quebec | 2008-2011 | Passivated canisters (24 hr) | All | 55 | — | 285 | 1.6 | 15.4 | Goldberg et al. 2015 |
First Nations reserve, Manitoba | 2011 | Passivated canisters (7 day) |
Winter | 20 | — | 53 | 1.2 | 11.1 | Weichenthal et al. 2013 |
Montreal, Quebec (Day-care centres) |
2008 | Passivated canisters (6 hours) |
Winter | 21 | — | 81 | 1.8 (GM) |
St-Jean 2012 | |
Sioux Lookout Zone, Ontario | 2017-19 | TD tubes (5 days) |
Winter | 98 | S and NS | 98 | 1.6 | 6.3 | Kovesi et al.2022; Health Canada 2021a |
Nunavik | 2017-18 | TD Tubes (7 days) | Winter and Spring | 54 | — | 54 | 0.4–0.7 | Personal communication, NRCC 2021 | |
Northeastern British Columbia | 2019 | TD tube (7 days) | Spring and Summer | 85 | — | 85 | 0.8 | 7.4 | Caron-Beaudoin et al. 2022 |
Across Canada | 2012-13 | TD tube (7 days) | All | 3524 | S and NS | 3524 | 1.1 | 7.2 | Li et al. 2019 |
Quebec City, Quebec | 2005 | 3 M organic vapor monitors (7 days) |
Winter | 94 | S and NS | 94 | 1.2 | Héroux et al. 2008 | |
Ottawa, Ontario | 2002-2003 | TD tubes (100 min) | Winter | 75 | S and NS | 75 | 2.2 | 5.2 (90th %ile) |
Zhu et al. 2005 |
Overall range | 0.4–2.2 | 2.4-18.6 | |||||||
OUTDOOR | |||||||||
Edmonton, Alberta | 2010 | Passivated canisters (7 days × 24 hours) |
Summer Winter |
50 50 |
— | 324 332 |
0.4 0.7 |
1.7 2.7 |
Health Canada 2013b |
Halifax, Nova Scotia | 2009 | Passivated canisters (7 days × 24 hours) |
Summer Winter |
50 50 |
— | 324 287 |
0.3 0.6 |
0.7 1.2 |
Health Canada 2012 |
Regina, Saskatchewan | 2007 | Passivated canisters (24 hours) |
Summer Winter |
111 106 | — | 108 95 |
0.2 0.6 |
0.6 2.0 |
Health Canada 2010a |
Windsor, Ontario | 2005-2006 | Passivated canisters (5 days × 24 hours) | Summer Winter |
46 47 |
— | 430 415 |
0.7 0.8 |
1.9 1.6 |
Health Canada 2010b |
Ottawa, Ontario | 2014 | Passivated canisters (48 hours) | Winter | 33Footnote c | NS | 127 | 0.6 | 1.1 | Mallach et al. 2017 |
Ottawa, Ontario (Newly built homes) |
2019-21 | Passivated canisters (96 hours) | All | 18 | — | 17Footnote d 13Footnote e | 0.3 0.3 |
1.3 2.2 |
Personal communication, Health Canada 2021a |
Montreal, Quebec | 2008-2011 | Passivated canisters (24 hr) | All | 55 | — | 200 | 0.6 | 1.5 | Goldberg et al. 2015 |
Ottawa, Ontario | 2002-2003 | TD tubes (100 min) | Winter | 74 | S and NS | 74 | 0.3 | 2.4 (90th%ile) |
Zhu et al. 2005 |
Overall range | 0.2–0.8 | 0.6–2.7 | |||||||
PERSONAL | |||||||||
Windsor, Ontario | 2005 | Passivated canisters (5 days × 24 hours) | Summer Winter |
45 48 |
— | 207 225 |
1.6 1.5 |
9.2 4.6 |
Health Canada 2010b |
Montreal, Quebec | 2009-2010 | Passivated canisters (10 days × 24 hours) | All | 72 | — | 647 | 2.1 | 3.7(75th%ile) | Smargiassi et al. 2014 |
Overall range | 1.5–2.1 | 4.6–9.2 | |||||||
GM, geometric mean; %ile, percentile; S, people who smoke; NS, people who do not smoke.
|
The distribution of indoor benzene concentrations by season in studies conducted by Health Canada in four cities is presented in Figure 1. It should be noted that for the studies in Edmonton, Halifax, and Windsor, multiple measurements were made at each home and these values have been averaged to present one value per home, while for the Regina study a single measurement was made at each home. Strong seasonal differences were not observed.
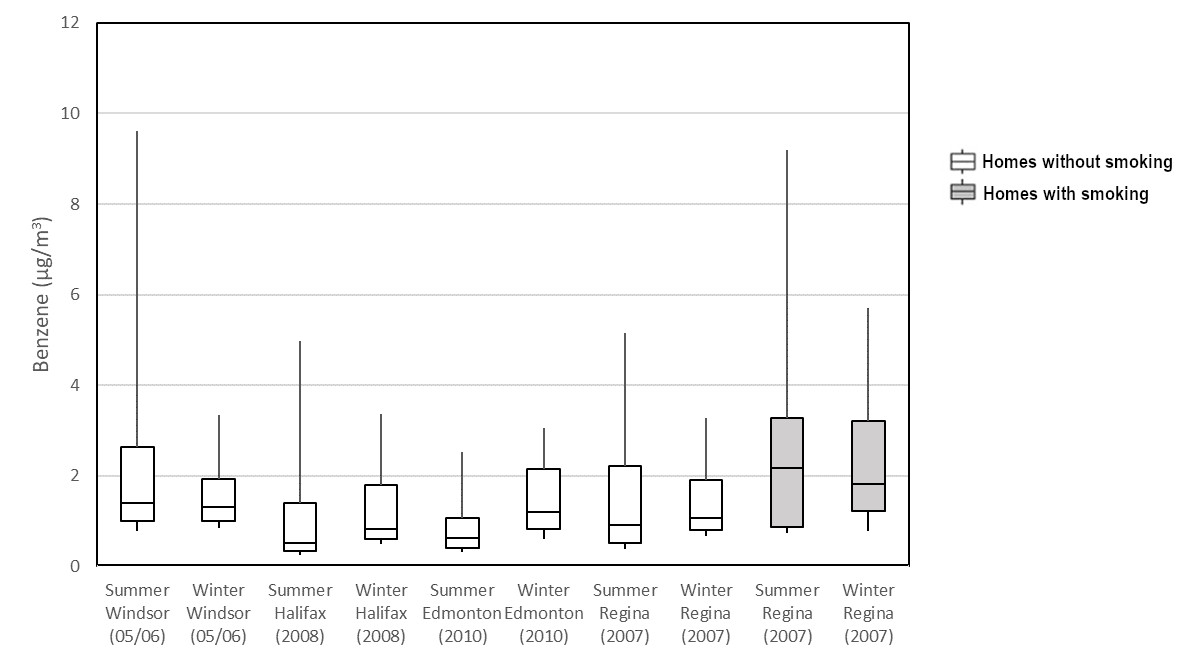
Figure 1 - Text description
A boxplot with benzene concentration (μg/m3) on the x-axis and identifiers of datasets (study season, location, and year) on the y-axis. The 75th, 50th, and 25th percentiles are represented by the top, middle, and bottom of the boxes. The whiskers represent the 90th and 10th percentiles. Plots are shown for smoking and non-smoking homes.
Figure 1. The 75th, 50th, and 25th percentiles are represented by the top, middle, and bottom of the boxes. The whiskers represent the 90th and 10th percentiles. Source data: Health Canada (2010a, 2010b, 2012, 2013b).
2.3 Indoor/outdoor ratios
An indoor-to-outdoor (I/O) ratio compares levels of benzene measured inside a given home to levels measured directly outside the same home. The distribution of I/O ratios for homes in four Health Canada studies is presented in Figure 2. Median I/O ratios of benzene in these four Canadian cities range from 1.3 to 7.9, indicating a predominance of indoor sources, particularly in homes with smoking. In a Health Canada study conducted in 33 Ottawa homes with attached garages, the median I/O ratios for benzene in homes with the garage fan off and the garage fan on were 2.8 and 1.6, respectively (Mallach et al. 2017).
In European studies, the I/O ratio is approximately 0.8, indicating that the main source of indoor benzene is from the outdoors. In North American studies, an overall I/O ratio of 0.12 was reported (Sekar et al. 2019), which is lower than that found in Health Canada residential studies. The studies included in these calculations cover a variety of locations, such as industrial and high traffic areas, in addition to residential environments, in Canada, the US and Mexico.
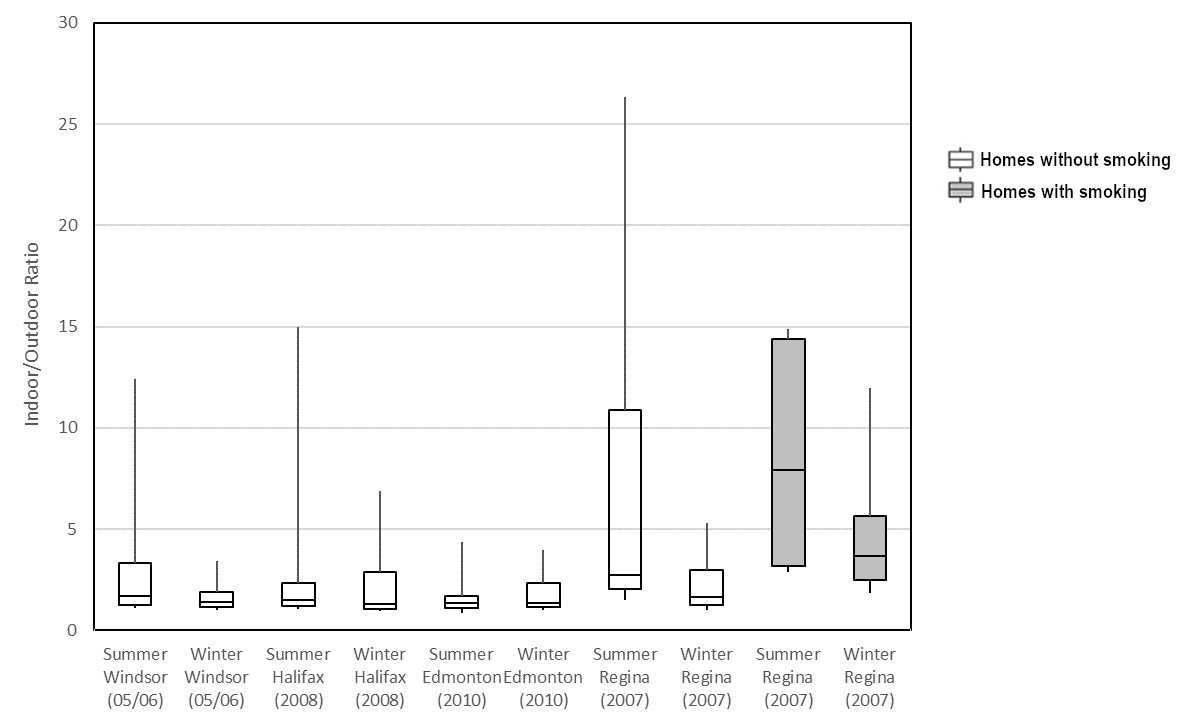
Figure 2 - Text description
A boxplot with the indoor/outdoor ratio of measured benzene concentrations (no units) on the x-axis and identifiers of datasets (study season, location, and year) on the y-axis. The 75th, 50th, and 25th percentiles are represented by the top, middle, and bottom of the boxes. The whiskers represent the 90th and 10th percentiles. Plots are shown for smoking and non-smoking homes.
Figure 2. The 75th, 50th, and 25th percentiles are represented by the top, middle, and bottom of the boxes. The whiskers represent the 90th and 10th percentiles. Source data: Health Canada (2012a, 2010b, 2010d).
3.0 Sources in the air
This section focuses on sources of benzene in outdoor and indoor air. While exposure to benzene can result from sources that contribute to media other than air (such as drinking water and food), these media are beyond the scope of this document.
Benzene is a natural component of petroleum products, such as crude oil and gasoline, and is added to unleaded gasoline at a rate of approximately 1% to enhance performance (ATSDR 2007; Health Canada 2013a; IARC 2018). It is also produced by combustion (natural and anthropogenic) and other anthropogenic sources. The primary industrial use of benzene is the manufacture of organic chemicals (including ethylbenzene, styrene, phenol, cumene, cyclohexane, nitrobenzene, alkylbenzene and chlorobenzene) which are used in the manufacture of plastics, resin, nylon, synthetic fibres, lubricants, rubbers, dyes, detergents and pesticides (ATSDR 2007; OEHHA 2014). Forty-three million tonnes of benzene were produced globally in 2012. A total of 700,000 tonnes were produced in Canada in 2019 (Umweltbundesamt 2020; Statistics Canada 2022).
3.1 Outdoor sources
When released to the environment, benzene is expected to partition to the air and surface water due to its relatively high vapour pressure, moderate-to-high water solubility and low octanol-water partition coefficient (Health Canada 2013a). According to Canada's National Pollutant Release Inventory (NPRI), 582 tonnes of benzene were released to the air from all sectors in 2020 (NPRI 2022). National benzene emissions declined 67% from the late 1990s to the late 2000s as a result of government intervention in the transportation and industrial sectors (Health Canada 2013a). Since 2006, approximately 500–800 tonnes of benzene are reported to be released to air per year (NPRI 2022).
Outdoor benzene concentrations vary seasonally in Canada, with higher concentrations observed in the winter months in urban and semi-rural locations (Kalenge et al. 2013; Miller et al. 2012). Land-use regression studies estimate that vehicle emissions are the source of 40%–65% of the benzene emissions in Canada (Stroud et al. 2016). The key predictors of outdoor concentrations of benzene in major cities are the length of nearby major roads, expressways and highways; the distance from major roads; and the number of and distance from industrial emission sources (Hystad et al. 2011; Wheeler et al. 2008; Amini et al. 2017). Benzene is released from gas stations via evaporation during normal operations and short-term emissions released during tanker truck fuel delivery (Health Canada 2023) and gasoline leaks from underground storage tanks (ATSDR 2007). Industrial sources in Canada that contribute to outdoor benzene levels include petroleum processing, automotive industry, chemical waste disposal, pulp and paper, and metal smelting (Xiong et al. 2020; Stroud et al. 2016; Miller et al. 2011; Levy et al. 2014; Hoffman et al. 2017). Wildfires and residential wood burning can also contribute significantly to outdoor benzene concentrations (Setton et al. 2015; Bari and Kindzierski 2017; Wentworth et al. 2018). In major cities, traffic has the greatest influence on benzene levels, but in rural areas, such as the Prairies, the petrochemical industry and other sources dominate (Stroud et al. 2016).
3.2 Indoor sources
A regression analysis was performed on the results of questionnaires on occupant behaviour and housing characteristics administered in Health Canada studies conducted in Edmonton, Halifax, Regina, and Montreal (Health Canada 2021a). The statistically significant multivariate modelling results are summarized in Table 3. Based on this analysis, key factors associated with indoor benzene concentrations in residences include the presence of an attached garage; storage of paints, solvents, and gasoline in the garage; storage of paints or solvents in the home; indoor smoking; infiltration from ambient air; and ventilation. While these models explain some of the variability associated with benzene concentrations (R2=0.14–0.64), a significant amount of variability remains unexplained, suggesting that other factors also influence indoor benzene concentrations. All of these studies measured indoor and outdoor air contaminants in detached single-family homes in and around major Canadian cities. The results may not be representative of all types of homes in Canada. The findings presented in Table 3 are represented as the relative (that is, fold) change in mean indoor benzene concentrations.
A multivariate regression analysis of findings from a small study conducted in a First Nations community in Sioux Lookout Zone indicated that higher indoor benzene concentrations were associated with the presence and number of people who smoke in the household, and the use of wood as a fuel source. Lower indoor benzene concentrations were associated with the use of an electric heating system, an intact building envelope, use of an HRV, and continuous ventilation (R2= 0.10–0.05) (Health Canada 2021a).
City | Edmonton | Edmonton | Halifax | Halifax | HalifaxFootnote 1 | HalifaxFootnote 1 | Regina | Regina | Montreal |
---|---|---|---|---|---|---|---|---|---|
Season | Summer | Winter | Summer | Winter | Summer | Summer | Summer | Winter | All year |
Study | Health Canada (2013b) | Health Canada (2013b) | Health Canada (2012) | Health Canada (2012) | Health Canada (2012) | Health Canada (2012) | Health Canada (2010a) | Health Canada (2010a) | Goldberg et al. 2015 |
Number of homes | 52 | 48 | 51 | 50 | 19 | 19 | 103 | 74 | 49 |
Sample size | 326 days | 300 days | 304 days | 285 days | 117 days | 110 days | naFootnote 2 | naFootnote 2 | 261 days |
R2 (coefficient of determination) | 0.49 | 0.59 | 0.46 | 0.36 | 0.43 | 0.26 | 0.46 | 0.64 | 0.14 |
Predictors | |||||||||
Any indoor cigarette smoking since last visit | 2.936 | 1.833 | |||||||
Any paints or solvents kept in home | 1.327 | ||||||||
Presence of attached garage | 1.981 | 2.018 | 2.635 | 2.039 | 3.426 | 2.263 | |||
Gasoline stored in attached garage | 4.949 | ||||||||
Paints or solvents stored in attached garage | 4.334 | ||||||||
Mean indoor AER (h-1) | 0.620 | 0.904 | 0.647 | ||||||
10% increase in outdoor benzene concentration (μg/m3) | 0.492 | 0.591 | 0.572 | 0.604 | 0.652 | 0.419 | |||
Window open since the last visit (1 window) | 0.963 | ||||||||
Window open since the last visit (any) | 0.583 | ||||||||
|
3.2.1 Attached garages
An important source of exposure to benzene indoors is the presence of an attached garage (Health Canada 2013a; Wheeler et al. 2013; Mallach et al. 2017; Cakmak et al. 2021; Gordian et al. 2010; Batterman et al. 2014). Benzene concentrations are significantly higher in garages than inside the home or outdoors, and garage sources are estimated to account for 40%–65% of the indoor benzene exposure (Health Canada 2013a; Mallach et al. 2017). Temperature and air pressure gradients often favour air migration from a colder, higher-pressure garage into the warmer, lower pressure home through connecting doors and gaps in the building envelope (Health Canada 2013a; Mallach et al. 2017). Garage sources of benzene include both vehicle exhaust (combustion) and evaporative emissions from gasoline and other products (Health Canada 2013a; Mallach et al. 2017). Canadian homeowners generally use their garage to park vehicles and store items such as automotive products, gas-powered equipment, and solvents (Mallach et al. 2017). Many of these items have been shown to release benzene in chamber tests, even when properly sealed and not in operation (Won et al. 2015).
Presence and characteristics of attached garages
When the benzene concentrations measured in homes in Health Canada studies in Windsor, Regina, and Halifax were stratified by garage type, it was found that the levels were higher in homes with attached garages than in those with detached garages or no garage (Health Canada 2013a). A regression analysis of data from homes in Edmonton, Halifax, Montreal, and Regina showed that the presence of an attached garage is a strong predictor of indoor benzene levels, regardless of season (benzene was 98%–243% higher in homes with an attached garage) (Health Canada 2021a). Using data from the CHMS, Wheeler et al. (2013) identified "garage on the property" as a predictor of higher levels of benzene and found that mean levels of benzene in homes with an attached garage were approximately double those in homes with a detached garage. Benzene concentrations were also significantly higher in homes with a connecting door to the garage compared to homes without a connecting door (Wheeler et al. 2013; Cakmak et al. 2021). Similar results were found in a study of three cities in the US (Batterman et al. 2014).
A Health Canada study of 33 homes in Ottawa with attached garages found that the median garage-to-outdoor (G/O) ratio for benzene was 11.4, indicating the presence of garage sources (Mallach et al. 2017). The G/O ratio was significantly lower (3.0) when an exhaust fan was operating in the garage. Operation of a fan in the garage also significantly reduced the median I/O ratios of benzene from 2.8 to 1.6 in addition to reducing the indoor concentration of benzene by 62%.
The median garage-to-indoor (G/I) ratio for benzene in the Ottawa study was 4.1 (Mallach et al. 2017), whereas a study conducted in 15 homes in Michigan reported G/I ratios that exceeded 10 (Batterman et al. 2007). The lower G/I ratios in the Health Canada study compared to the US study were attributed to increased stack and wind forces during winter sampling, which promote the transfer of air from the attached garage into the home, in comparison to the spring-summer sampling done in Batterman et al. (2007). In the Ottawa study, there were significant differences in the outdoor-corrected garage benzene concentrations and the garage AER between "leaky" and "tight" garages; however, homes with leakier garages (in other words, garages with a higher rate of air exchange per hour) did not have significantly lower levels of benzene in the home. The lack of statistically significant findings may be a result of the relatively small sample size used in that study, given that the overall trends in the data suggest lower indoor levels of pollutants in homes with leakier garages (Mallach et al. 2017).
In previous studies, the placement of an attached garage and the location of parked vehicles were found to influence indoor benzene levels. Garages attached to the ground floor have a greater impact on indoor benzene than subterranean garages; however, living areas located directly above a garage have higher indoor benzene levels that those located adjacent to a garage. Mean indoor benzene levels were approximately 2-fold higher when a car was kept near the house, in an attached garage or carport (Health Canada 2013a).
Product storage in attached garages
Data from Health Canada studies indicate that the storage of gasoline, paints and solvents in attached garages had a significant impact on indoor benzene concentrations in homes in Halifax (Health Canada 2021a). Storing gasoline or paints/solvents in the garage was associated with higher benzene levels in Halifax homes in summer, where mean benzene levels increased by 395% for gasoline and 333% for paints/solvents (Health Canada 2021a).
A Canadian study on chamber tests of VOC emissions from evaporative sources in residential garages found that gasoline-related products were high emitters of BTEX (benzene, toluene, ethylbenzene, and xylenes) species (Won et al. 2015). Gas-powered products that emitted high levels of benzene included snow blowers, lawn mowers, lawn trimmers, and chain saws. Equipment was run for 30 minutes before being shut off, wiped off, and placed in an emission testing chamber. Older products (including a snow blower, lawn mower, and lawn trimmer purchased in 2002 or 2003) had benzene emission factors that were 6 to 13 times higher than the same type of equipment purchased in 2014. For example, the older snow blower had a benzene emission factor of 1285 μg/h compared to 98 μg/h for the newer machine. Another high emitter of benzene was a 5-L regular grade gasoline container; with the cap closed, the benzene emission factor was 1241 μg/h at 23°C. Benzene emission factors for the gas container varied with the test temperature: at 14°C and at 4°C, emission factors were 50% and 70% lower, respectively; at 30°C, the emission factor was 20% higher. Low emission factors (that is, benzene emission factors of less than 0.6 μg/h) were obtained for paint products, an adhesive product, a degreaser, automotive products, wax or polishes, lubricant, lawn and plant care products, roof products, and driveway products. However, one of the paint products had a benzene emission factor that was 40 times higher at 30°C than at 23°C, suggesting that individual product composition may play a role (Won et al. 2015).
Benzene concentrations were modelled for the garage and home using the emission factors for these garage products. In the garage, the gasoline containers and gas-powered equipment were the main sources of benzene, with larger relative contributions from older equipment (compared to new equipment). Older gasoline-powered equipment is expected to increase benzene concentrations in the garage 2-fold, compared to newer equipment. Other products, such as degreaser, paint remover, and adhesive were identified as minor sources of garage benzene. The dominant source in the house was infiltration from the attached garage. Seasonal differences between predicted house and garage concentrations were minimal, which is consistent with the seasonal indoor benzene levels seen in Health Canada studies (Won et al. 2015).
In a study of over 500 homes with attached garages in Alaska, the indoor benzene concentration was positively associated with the presence of portable gasoline containers in the garage (Gordian et al. 2010). A strong correlation was reported between the benzene concentration and the number of engines stored in the garage (small engines plus vehicles). In a study of homes in Detroit, gas-powered equipment was stored in the basement of over 30% of homes, as most of the homes did not have an attached garage. The authors estimated that at the median indoor benzene concentration, 8% of the contribution was from the basement; however, in homes with higher benzene levels, up to 40% of indoor benzene was from the basement (Du et al. 2015).
3.2.2 Smoking
Health Canada studies show that smoking in the home is the third strongest determinant of indoor benzene levels, after attached garages and storage of gasoline, paint, and solvents. Higher levels of benzene have been reported in the homes of people who smoke, compared to those where no smoking occurs (Health Canada 2013a; Li et al. 2019; Zhu et al. 2013; Wheeler et al. 2013; Chin et al. 2014; Schlink et al. 2010; Herberth et al. 2014). A multiple regression analysis (Health Canada 2021a) showed that indoor cigarette smoking was a strong predictor of mean indoor benzene levels in Regina in both summer and winter, with increases of 194% and 83%, respectively. Similarly, the presence of smokers in the home was associated with a 56% increase in mean benzene levels in a study of First Nations homes in Sioux Lookout Zone (Health Canada 2021a).
Higher mean levels of benzene were observed in smoking homes compared to non-smoking homes in the 2012 to 2013 Cycle of the CHMS (Cycle 3) (Li et al. 2019) as well as between 2009 and 2011 (Cycle 2) (Zhu et al. 2013). Using data from CHMS Cycle 2, Wheeler et al. (2013) identified regular smoking in the home as a strong predictor of higher benzene concentrations and reported a 136% increase in concentrations for this predictor. Similarly, studies in the US and Europe have shown that smoking or exposure to environmental tobacco smoke is associated with higher levels of benzene in the home (Chin et al. 2014; Schlink et al. 2010; Herberth et al. 2014).
Benzene emission factors may range from 296 to 610 μg per cigarette. Most benzene emissions from cigarettes result from sidestream smoke (in other words, smoke from the lit end of a cigarette) (Health Canada 2013a). Third-hand smoke, which is carried on clothing and re-emitted into indoor air, is also an important source of benzene from cigarette smoke. A recent study of moviegoers in Germany reported that in a non-smoking theatre, occupants were exposed to VOC emissions (including benzene) equivalent to 1–10 cigarettes of second-hand smoke, which was carried on clothing (Sheu 2020). In addition to cigarette smoke, benzene has been identified in emissions from little cigars and cigarillos, e-cigarettes, heated tobacco products, and hookah smoke (Pickworth et al. 2018; Logue et al. 2017; Cancelada et al. 2019; Kassem et al. 2014). No studies were identified about the extent to which these products contribute to indoor benzene concentrations.
3.2.3 Infiltration from ambient air
The influence of ambient benzene levels on indoor levels is highly variable, reflecting variation in ambient benzene both spatially and temporally (Health Canada 2013a). As described in Section 3.1, benzene in ambient air comes from vehicle emissions, evaporative emissions from gasoline, industrial emissions, and wood burning. Multiple regression analysis showed that a 10% increase in outdoor benzene concentration was associated with increases of approximately 4%–6% in mean indoor benzene levels in Edmonton (4.8% in summer and 5.8% in winter), Halifax (5.6% in summer and 5.9% in winter), Regina (6.4% in winter), and Montreal (4.1%, all seasons) (Health Canada 2021a). Correlations between indoor and outdoor benzene concentrations were not tested for First Nations homes in Sioux Lookout Zone. However, several indicators of a secure building envelope which would prevent infiltration (such as sealed building services, vinyl window frames or siding, and extended downspouts) were associated with a 28%–49% lower indoor concentration of benzene (Health Canada 2021a).
The presence of benzene indoors may be partially explained by infiltration of outdoor vehicle combustion sources. In a study of four schools in Ottawa, MacNeill et al. (2016) showed that the indoor concentration of benzene decreased by 22%–42% when the ventilation schedule was altered so that high ventilation periods did not coincide with rush hour traffic. In a study of homes in Detroit, the amount of traffic within either 100 or 300 m of a home was only weakly associated with indoor benzene (Chin et al. 2014). However, the results of a study of 20 daycare centres in California showed that indoor benzene was correlated with proximity to traffic (Hoang et al. 2017). A study in Germany showed that benzene levels were higher in homes adjacent to heavy traffic roads (Schlink et al. 2010). A recent review reported that, on average, outdoor benzene mainly from traffic emissions could account for 84% of the indoor concentration of benzene in developed countries and regions (Liu et al. 2020). Indoor benzene levels were found to be influenced by proximity to busy roads and gasoline stations in a study conducted in Greece (Vardoulakis 2020).
Limited evidence on the impact of outdoor benzene sources such as gasoline stations and industrial activity on indoor benzene levels was identified. Indoor and outdoor air levels of benzene are higher near sources of emissions such as filling stations (WHO 2000). These releases are from day-to-day evaporative losses during the operation of the gasoline station, as well as intermittent gasoline vapour releases during filling of underground storage tanks. In a recent Health Canada assessment (Health Canada 2023), the long-term outdoor benzene concentration attributable to evaporative loss from a gas station was modelled for residences at different distances. At a distance of 20 m from the gas station fence line, the modelled air concentrations were 0.88, 3.5, and 9.2 μg/m3 for baseline, moderate, and high-throughput scenarios, respectively. The concentrations decreased with increasing distance from the gas station, but only reached a 1 × 10-6 cancer risk level of 0.29 μg/m3 at distances greater than 70, 160, or 300 m for the baseline, moderate, and high-throughput scenarios, respectively. Short-term increases in benzene concentration due to tank refilling were also modelled for residential areas near gas stations. In this assessment, the assumption was made that the indoor air concentration of benzene resulting from gasoline station emissions is equal to the modelled outdoor air concentration.
Caron-Beaudoin et al. (2022) suggested a possible correlation of indoor air benzene level with proximity of the home to fracking operations (distance and density of wells). However, indoor benzene levels in the study were similar to those in a nationally representative sample (that is, CHMS).
3.2.4 Building materials and consumer products
Storage of paints or solvents in the home was a predictor of indoor benzene levels in Regina in winter (Health Canada 2021a). However, the previous Health Canada Guidance (Health Canada 2013a) stated that no associations were observed between elevated benzene levels and recent renovations or new products in Health Canada studies conducted in Halifax, Quebec City, and Regina. Moreover, in the Quebec City study, new carpet and recent renovations were associated with lower benzene levels, although this may be due to secondary factors such as sample size (Health Canada 2013a). Studies conducted in the US and Europe indicated that building materials, renovations, or use of paints or adhesives did not contribute significantly to indoor benzene levels (Health Canada 2013a). Similarly, regression analysis of data from Edmonton, Regina, Halifax, and Montreal did not show an association between indoor benzene levels and painting, finishing or varnishing work done in the past year.
Studies based on indoor air measurements and activity surveys for the CHMS showed that major renovations done in the past month did not increase the level of benzene in the home (Wheeler et al. 2013; Cakmak et al. 2021). In addition, the use of stains and paints as well as new carpet were not identified as predictors of indoor benzene concentration (Cakmak et al. 2021). Preliminary data from an in-progress Health Canada study suggest that benzene levels in recently built homes fall in the same range as those measured in other Canadian homes (see Table 2; Health Canada 2021a).
In older studies, benzene was detected in some building products (painted drywall, adhesives, caulking, and insulation) in Canada and the US (Health Canada 2013a), but the emission factors were generally low. Similar results were obtained in more recent studies. In a series of chamber emission tests for new building products, benzene was detected in 14%–65% of tested materials; however, most emission factors were low (Won et al. 2014, 2015; Health Canada and National Research Council Canada 2019). The lowest emissions (<2.5 mg/m2/hour) were associated with dry materials, such as vinyl flooring and wood-based materials, and higher emissions with insulation materials (up to 4.2 mg/m2/hour), caulking and foam sealants (0.4–1 200 mg/m2/hour). In one study, benzene was not detected in paint materials above the method detection limit but was detected in 43% of paints at levels below the method detection limit (that is, above zero) (Won et al. 2014). Air concentration modelling in this study indicated that paint was the main source of benzene in indoor air, despite the lower emission factors, likely due to the large surface area of paint applied on walls and ceilings. The model did not include sources from an attached garage (as described in Section 3.2.1.) and 1-year mean modelled concentrations of benzene were an order of magnitude lower than levels identified in the literature, indicating that the materials tested are likely not major sources of benzene indoors. In a later modelling study on products stored in attached garages, modelled benzene concentrations were consistent with the values reported in the literature (Won et al. 2015). This study reported that paint, thermal insulation, and I-beam joists may be important secondary indoor sources of benzene, after attached garages.
Although the previous Health Canada Guidance (Health Canada 2013a) included several international studies in which benzene was detected in a small number of household or consumer products, indoor benzene concentrations were generally not associated with product use (Health Canada 2013a). No association was identified between benzene concentration and use of candles, incense, air fresheners or cleaning products in a regression analysis of data from homes in Edmonton, Regina, Halifax and Montreal (Health Canada 2021a). Similarly, in the CHMS (Cakmak et al. 2021) candle use and hobby activity were not predictors of indoor benzene concentration in non-smoking homes. However, using data from all homes in the CHMS, Wheeler et al. (2013) identified a positive association between model-making activity in the previous three months and a 31% increase in log-transformed benzene concentrations, and a non-significant association between use of candles in the previous week and a 12% increase in log-transformed benzene concentrations.
Other modelling studies have shown that indoor air benzene concentrations may increase during use of petroleum-based solvents and burning of incense or candles (Hollins et al. 2013; Manoukian et al. 2016; Petry et al. 2014). In Korea, benzene has been detected in consumer products, such as insect repellents, glue, correction fluid, and pens (Lim et al. 2014; Rahman and Kim 2014). One study conducted in Korean homes with children showed higher benzene levels in homes where air fresheners had been applied (Lee et al. 2014). There is no data to indicate that the presence of benzene in these products contributes to increased indoor air concentrations of benzene in Canadian homes.
3.2.5 Other sources
Wood and other heating sources
Wildfires and wood smoke are a source of outdoor benzene. The use of wood or other heating sources, such as oil or electrical, were not found to be associated with residential indoor benzene concentrations in Health Canada studies conducted in Edmonton, Halifax, Montreal and Regina (Health Canada 2021a). However, in First Nations homes in Sioux Lookout Zone, the use of wood as a main heating system was associated with a 53% increase in benzene concentrations and use of electrical heating was associated with a 48% decrease. International studies have reported that benzene may be emitted from wood-fired hydronic heaters and decorative ethanol fireplaces (Aurell et al. 2012; Schripp et al. 2014). Although benzene is emitted from wood-burning appliances, evidence obtained prior to the publication of the previous Health Canada Guidance did not suggest that wood-burning appliances are a source of elevated indoor benzene levels (Health Canada 2013a). No recent publications on the association between the use of wood-burning appliances and indoor benzene levels were identified. Overall, it is thought that properly installed and maintained wood-burning appliances vent pollutants to the outdoors and do not contribute to indoor benzene levels (Health Canada 2013a).
Vapour intrusion
In Canadian residential areas where there is benzene-contaminated groundwater or soil, migration of benzene through unsaturated soil and into homes may potentially occur (vapour intrusion). In First Nations homes in Sioux Lookout Zone, non-significant associations were found between higher indoor benzene concentrations and nearby standing water, or the presence of a dirt floor in the basement (Health Canada 2021a). Accidental releases of benzene may occur at any stage in the production, storage, use and transport of benzene, crude oil and gasoline (Health Canada 2013a).
The rate of vapour intrusion of VOCs from contaminated water sources depends on many factors. Simulations of vapour intrusion scenarios indicate that, in addition to the concentration of the contaminant in the source water, important factors include the distance and relative direction (up- or down-gradient) from the source, the presence of impervious surfaces (such as paving), the depth of the region of aeration above the water table, and the depth and location of breaches in the building envelope (such as foundation cracks) (Dehate et al. 2011; Mustafa et al. 2014; Yao et al. 2011). A simulation of benzene transport from a contaminated aquafer at a concentration as low as 5 μg/L resulted in benzene breakthrough at up to 1 000 m from the source. At a distance of 43 m from the source, it took at least two years for the air to be restored to a concentration that met a cancer-based guidance level (3.1 μg/m3) (Mustafa et al. 2014). In a study of a community in Ohio, indoor benzene levels were 2 to 17 times higher than the guidance level (3.1 μg/m3) in three of four homes situated less than 2 km from a natural gas compressor station (Martin et al. 2021). In a study of commercial, single and multi-family residential properties located near three former manufactured gas plants, benzene was among the most frequently detected chemicals in indoor air. Cancer risks for benzene in this study ranged from acceptable (10-6) to below acceptable (10-4) risk levels (Dehate et al. 2011).
Contaminated drinking water
If benzene is present in the domestic water supply, volatilization from water while bathing, showering, or running faucets may occur. Note that contamination of water with benzene is extremely rare in Canada. For more information on this route of exposure, readers can refer to Health Canada's Guidelines for Canadian Drinking Water Quality (Health Canada 2013a; Health Canada 2009).
3.3 Ventilation
Ventilation is frequently represented by AER, reported as air changes per hour (h-1). Ventilation depends on multiple factors, such as home construction, use of exhaust fans, geographic location, season, and the extent to which windows and doors are kept open (Health Canada 2013a). AER is influenced by indoor/outdoor temperature differences and by outdoor wind speeds, which can increase infiltration or exfiltration of air through small openings in the building envelope. Homes in colder climates and newer homes tend to be built more tightly than older homes, or those in other climates (Health Canada 2013a). A higher ventilation rate is generally expected to lower concentrations of indoor contaminants; however, it can also cause negative effects such as dryness, drafts, or irritation due to the sound of exhaust fans.
In Health Canada studies, open windows in summer were associated with a 42% decrease in benzene concentration in homes in Regina and a 4% decrease in Edmonton. A higher mean AER was also associated with significantly lower indoor benzene concentrations in Halifax in summer and winter, and in Edmonton in the summer (a 10%–38% decrease in benzene concentration with each AER increase of 1 h-1). Using data from the CHMS, Wheeler et al. (2013) also identified "open windows on typical day in past week" as a predictor of lower benzene levels in homes (21% decrease in log-transformed benzene concentration). Continuous ventilation and the use of an HRV were associated with lower benzene levels in First Nations homes in Sioux Lookout Zone (36% and 47% decrease, respectively) (Health Canada 2021a).
Studies in the US have also shown that the indoor benzene concentration is inversely related to AER, and personal exposure to benzene is inversely related to open windows (Hoang et al. 2017; Symanski et al. 2009). As described in Section 3.2.1, increased ventilation in an attached garage (installation of an exhaust fan) also significantly reduces the concentration of benzene in the home.
4.0 Toxicokinetics
4.1 Absorption, distribution, metabolism, and excretion
The toxicokinetics of benzene have been studied extensively and are described in detail in OEHHA (2014) and ATSDR (2007). The following paragraphs summarize these reviews.
Benzene is rapidly absorbed by humans following inhalation, which is the major route of human exposure. Studies of human inhalation exposure to concentrations ranging from 1 to 100 ppm report that approximately half of the inhaled dose is absorbed (ATSDR 2007; OEHHA 2014). Absorption occurs rapidly in the first few minutes of exposure and decreases significantly thereafter (ATSDR 2007). Inhaled benzene is distributed throughout the body following absorption into blood. In rodents and dogs, benzene is detected in bone marrow, kidney, liver, brain and spleen. Benzene is preferentially distributed to lipid-rich tissues and relative uptake in tissues depends on the blood perfusion rate. In humans, benzene is distributed to a range of tissues. After lethal doses in humans, benzene was found in the blood, brain, liver, kidney, stomach, bile, and abdominal fat (ATSDR 2007). Benzene crosses the human placenta and has been measured in cord blood at levels equal to those in maternal blood (ATSDR 2007). Benzene metabolites are distributed widely to tissues and organs such as the kidney, lung, brain and bone marrow (ATSDR 2007; OEHHA 2014).
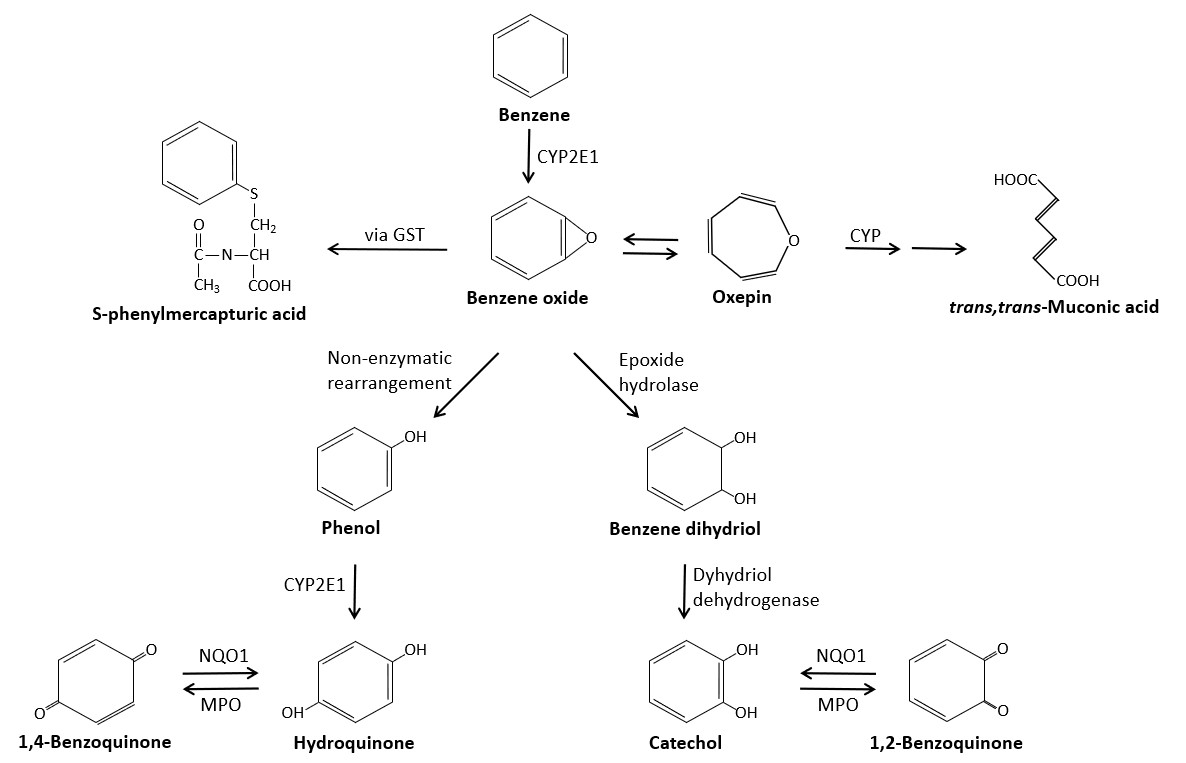
Figure 3 - Text description
Figure 3. A simplified metabolic scheme for benzene showing major pathways and metabolizing enzymes. Key pathways and metabolites shown include 1) benzene to benzene oxide to S-phenylmercapturic acid and trans,trans -muconic acid; 2) benzene oxide to phenol to hydroquinone to 1,4-benzoquinone; and 3) benzene oxide to benzene dihydriol to catechol to 1,2-benzoquinone.
Simplified metabolic scheme for benzene showing major pathways and metabolizing enzymes (modified from Rappaport et al. 2010). CYP2E1, cytochrome P450 2E1; CYP, cytochrome P450 enzymes; GST, glutathione S-transferase; NQO1, NAD(P)H:quinone reductase; MPO, myeloperoxidase.
Benzene toxicity is widely considered to be due to action of its metabolites (see Section 5.5.2). Figure 3 shows the major pathways of benzene metabolism. Metabolism occurs primarily in the liver, but may also occur to a lesser extent in the bone marrow. Cytochrome P450 component CYP2E1 (but also CYP1A1, CYP2B1, CYP2F1, and CYP2F2, to varying extents) catalyzes the oxidation of benzene to benzene oxide, which exists in equilibrium with oxepin (OEHHA 2014; ATSDR 2007). Benzene oxide is then metabolized via multiple pathways. In the predominant pathway, non-enzymatic rearrangement of benzene oxide produces phenol, which is then oxidized by CYP2E1 to hydroquinone (ATSDR 2007). Hydroquinone can then be oxidized to 1,4-benzoquinone via myeloperoxidase in bone marrow (ATSDR 2007). In an alternative pathway, benzene oxide is enzymatically transformed to benzene dihydrodiol, which is dehydrogenated to catechol (ATSDR 2007). In a third pathway, benzene oxide reacts with glutathione to form S-phenylmercuric acid (SPMA) (ATSDR 2007). In a final metabolic pathway, benzene oxide undergoes a ring-opening conversion to trans, trans-muconaldehdye, which is then oxidized to trans, trans -muconic acid (t,t-MA) (OEHHA 2014, ATSDR 2007). Benzene metabolites with hydroxyl groups (in other words, phenol, catechol, hydroquinone, and 1,2,4-benzenetriol) can form sulfates and glucuronidates and quinol thioethers after reaction with glutathione as part of phase II metabolism (OEHHA 2014). Single-nucleotide polymorphisms (SNPs) that affect the metabolic enzymes GSTT1 (glutathione-S-transferase theta-1), NQO1, CYP2E1, and epoxide hydrolase 1 (EPHX1) result in changes in the relative levels of the metabolites SPMA, phenol, catechol, and hydroquinone formed by the liver (OEHHA 2014).
Benzene is exhaled unchanged via the lungs and excreted as metabolites in the urine; small amounts are also excreted as parent compound. The rate and amount of excretion via the lungs are dependent on exposure dose and route. In one study of human volunteers exposed to 166–198 mg/m3 (52–62 ppm), approximately one third of absorbed benzene was exhaled unchanged (OEHHA 2014). Most of the catechol and phenol metabolites are excreted in urine within 24 hours, while hydroquinone requires 48 hours (OEHHA 2014). In animal studies, the relative amounts of benzene metabolites excreted in urine varied with the species and exposure concentration (OEHHA 2014). Some evidence suggests that the relative amounts of metabolites produced in humans also vary with the exposure concentration; however, considerable uncertainty remains in this regard (see Section 4.2).
4.2 Physiologically based pharmacokinetic modelling
Several physiologically based pharmacokinetic (PBPK) models have been developed to simulate benzene disposition in humans, mice and rats. All models include simulations of blood, fat, liver, lung and lumped compartments representing other slowly (such as skeletal muscle) and rapidly perfused tissues (such as kidneys, other viscera). Simulation of bone marrow, the primary target for benzene toxicity, is also included in several models. Flow-limited exchange of benzene is assumed to take place between blood and tissues, with excretion to exhaled air, and, in one model, breastmilk (ATSDR 2007). Metabolism, conjugation, and elimination are presented in most models as capacity-limited or first-order processes. Metabolites are assumed to be excreted in urine at a rate equal to their formation, or a first-order excretion rate constant is used. Most models attribute metabolic processes to the liver, and more recent models include bone marrow as a site of metabolism (ATSDR 2007). The models described here address benzene concentrations in the range of occupational or experimental exposures; no models were identified that addressed lower, environmental exposures (that is, <0.1 mg/m3).
Potential metabolic differences in the population have been predicted by PBPK models. Multiple models have predicted that at an exposure level of 80 mg/m3 (25 ppm) for 2 hours, human females metabolize up to 26% more benzene than males, for the same exposure scenarios, and that blood benzene levels are higher in males (Brown et al. 1998, as cited in ATSDR 2007 and OEHHA 2014). Model simulations showed that age-related changes in mouse physiology, including decreased elimination of hydroquinone conjugates at 18 months, were responsible for altered disposition of benzene in aged mice (McMahon et al. 1994, as cited in OEHHA 2014). A PBPK model applied to a range of benzene exposures and concentrations indicated that neonates and pregnant women metabolize benzene less efficiently than average adults, particularly at higher exposure concentrations (Valcke and Krishnan 2011).
Some PBPK models also predict metabolic differences based on exposure levels in animals and humans. Medinsky et al. (1989) developed a human model based on toxicological studies to predict metabolism at concentrations near 32 mg/m3 (10 ppm). Higher levels of hydroquinone and lower levels of t,t-MA were predicted for 8-hour exposures below 10 ppm. At concentrations above 10 ppm, detoxification metabolites such as phenyl conjugates are predicted to predominate. The authors proposed that lower exposures may cause preferential production of toxic metabolites (Medinsky et al. 1989; OEHHA 2014).
Multiple PBPK models have been developed to simulate the shape of the dose-response curve for relative levels of urinary metabolites resulting from air benzene concentration in occupational settings at higher (usually over 100 ppm, 320 mg/m3) versus lower levels (close to 1 ppm, 3.2 mg/m3).. Observations of the differential production of metabolites at lower exposures in a cohort of male and female workers in Tianjin, China led to the development of models to test whether benzene is metabolized by different pathways at different concentrations. Kim et al. (2006a, 2006b) reported that the urinary concentrations of benzene and five metabolites (phenol, t,t-MA, SPMA, hydroquinone, and catechol) were consistently elevated in this cohort when the median air benzene levels were between 0.64 and 6.4 mg/m3 (0.2–2 ppm), depending on the metabolite. Metabolite production reportedly declined 2.5- to 26-fold as the median air benzene levels increased from 0.086 to 49.3 mg/m3 (0.027–15.4 ppm), with increased production of hydroquinone and t,t-MA, in particular, at low exposures. Michaelis-Menten-like models were fitted to individual urinary benzene metabolites and the corresponding air concentrations of benzene (range: 0–975 mg/m3 [0 ppm to 299 ppm]). Based on these data, a low-affinity pathway, thought to be based on CYP2E1, was predicted to occur at a concentration of 981 mg/m3 (301 ppm) and a high-affinity pathway, that is predominant at less than 3.2 mg/m3 (1 ppm) (Rappaport et al. 2009, 2010). These models have been criticized based on a lack of measurement or incorporation of background concentrations and co-exposures, for their treatment of the control group data, and for their modelling of low-exposure doses (Price et al. 2012; McNally et al. 2017; Cox et al. 2017, Boogaard 2017). Although Rappaport et al. (2013) responded to several of these concerns, they have not provided a response to more recent comments.
An alternative to the low-affinity/high-affinity model was developed by Knutsen et al. (2013) and was validated using the same Tianjin cohort data. This model was based on a mouse PBPK model and incorporated bone marrow and urinary bladder compartments, human liver microsomal protein levels, CYP2E1, and metabolite-specific conversion rate parameters. The model predicted reasonably well fitted J-shaped curves for all five metabolites, three of which (phenol, hydroquinone, and catechol) crossed the y-axis. A more recent model, which is based on Bayesian analysis (Cox et al. 2021) and was developed using multiple datasets was reported to show a roughly linear correlation between air benzene concentrations below 15 ppm (48 mg/m3) and production of metabolites. The authors also report a J-shaped curve for the Tianjin worker data that is saturated above 10 ppm. These models correct several shortcomings of the two-pathway model but are also subject to some uncertainties. As with all models discussed here, development and validation are based on occupational exposure to benzene, with little to no metabolic data available for air concentrations below 0.5–1 ppm (1.6–3.2 mg/m3), which is considered a very low occupational exposure. Without data for low exposures, models cannot be validated below this range. Given the lack of certainty in this range, it is not possible to state which model (either the two-pathway model proposed by Kim and Rappaport, or the single-pathway models proposed by Knutsen and Cox) provides an accurate representation of pharmacokinetics at low concentrations (that is, <1.6 mg/m3, 0.5 ppm).
The lack of available data and models that address air benzene exposure at environmentally relevant concentrations (that is, <10 μg/m3, or levels that are typical of indoor residential exposure in Canada), constitutes a key data gap. A single study was identified that begins to address this issue. The body burden of t,t-MA relative to inhaled benzene at environmentally relevant concentrations was reported in gasoline pump workers, car drivers and an environmentally exposed control group in India (Majumdar et al. 2016). Estimated inhalation exposure levels for these groups were 137.5, 97.9 and 38.7 μg/m3, respectively, with urinary t,t-MA levels of 145.4 ± 55.3, 112.6 ± 63.5 and 60.0 ± 34.9 μg/g creatinine. (The studied populations rarely, if ever, consume sorbic acid and are not expected to have significant background levels of t,t-MA.) The authors report that the internal dose or body burden of t,t-MA is not proportional to the level of external exposure to benzene. The estimated body burden at the lowest concentration is slightly higher than would be expected from a strictly linear metabolic relationship. This, in turn, results in a slightly higher risk of cancer at lower exposure levels, and a slightly lower risk at higher exposure levels, than is predicted by a linear risk model.
4.3 Biomonitoring
Concentrations of benzene in blood were measured from 2012 to 2017 in Cycles 3 to 5 of the CHMS, a nationally representative biomonitoring survey. Urinary concentrations of benzene and its metabolites (t,t-MA and SPMA) were measured in Cycles 2 to 4 (2009–2015) but were discontinued in Cycle 5 (2016–2017) (Health Canada 2015, 2017, 2019a). Benzene levels in blood are considered a more sensitive and specific biomarker of recent benzene exposure. Urinary metabolites can be non-specific or represent only a fraction of benzene exposures (Hays et al. 2012; Arnold et al. 2013).
4.3.1. Blood benzene
Blood benzene concentrations were measured in CHMS participants aged 12 to 79 years in Cycles 3, 4, and 5 (Health Canada 2015, 2017, 2019a). Geometric mean (GM) benzene levels in blood ranged from 0.034 to 0.037 μg/L, with 95th percentiles of 0.20–0.24 μg/L. Biomonitoring data from the US National Health and Nutrition Examination Survey (NHANES) are also available for blood benzene. Median values were below the limit of detection (0.024 μg/L) for NHANES data from 2011 to 2016; the 75th percentile ranged from 0.027 to 0.045 μg/L, and the 95th percentile, from 0.25 to 0.29 μg/L (US CDC 2017).
In CHMS Cycles 3 and 4, blood benzene levels were significantly higher among people who smoke (median of 0.13 μg/L vs. 0.025 μg/L among people who do not smoke), and smoking was the strongest predictor of blood benzene. In biomonitoring studies conducted in the US, higher blood benzene levels are observed among people who smoke compared to those in the general population who do not smoke (US CDC 2017).. Among people who do not smoke, participant reports of exhaust odour in the home, presence of an attached garage, and recent use of mothballs or paint stripper were each significantly associated with blood benzene levels (Lyonnais-Gagnon et al. 2022). Living in urban areas and exposure to gasoline or petroleum products (for example, during vehicle refueling) were also associated with higher blood benzene in the US and in Italy (US CDC 2017). Additional univariate analyses of CHMS data suggested that South Asians in Canada have higher blood benzene concentrations than the general population (Lyonnais-Gagnon et al. 2022; Karthikeyan et al. 2022). Previous estimates have suggested that up to 99% of benzene exposure in Canada comes from the air (Health Canada 2009). However, CHMS-measured blood concentrations were 3.8- to 5.4-fold higher than those predicted by PBPK modelling (using the model developed by Brown [1998]) from indoor air measurements, suggesting that additional sources of benzene exposure, either from other air exposures, or other routes of exposure, have yet to be identified (Lyonnais-Gagnon et al. 2022).
Hays et al. (2012) used a PBPK model developed by Brown et al. (1998) to estimate a biomonitoring equivalent (BE) for blood benzene concentration at the United States Environmental Protection Agency (US EPA) non-cancer reference concentration (RfC) of 0.030 mg/m3. This model simulates the initial inhalation absorption and disposition of benzene in humans and was selected because of its simplicity, consistency with human kinetic data and sex-specific consideration of differences in body fat. The authors estimated a BE of 0.15 μg/L in blood, corresponding to the predicted steady state blood benzene concentration at 0.030 mg/m3. The GM blood benzene levels of 0.034 to 0.037 μg/L found in the Canadian general population in 2012–2017 are below the non-cancer BE. However, the GM blood benzene level of 0.14 μg/L found in people who smoke in Cycles 3 and 4 approaches the non-cancer BE, suggesting that this group is at risk of benzene-related health effects (Faure et al. 2020; Health Canada 2015, 2017, 2019a). Hays et al. (2012) also developed BEs corresponding to unit risk values for cancer developed by the US EPA (See Appendix D). Blood benzene GMs and medians for the general population in Canada from 2012 to 2017 are just above the range of BEs (0.0058–0.020 μg/L) corresponding to a cancer risk of 1 × 10 -5 (with a corresponding air concentration of 1.3 to 4.5 μg/m3). GM and median blood benzene levels are significantly higher for people who smoke, indicating an elevated risk of cancer (Faure et al. 2020; Health Canada 2015, 2017, 2019a).
4.3.2 Urinary benzene and metabolites
Benzene metabolites, t,t-MA and SPMA, were measured in urine in Canadians aged 3 to 79 years in CHMS Cycles 2, 3, and 4 (Health Canada 2015, 2017). As indicated above, conclusions based on concentrations of urinary metabolites of benzene should be made with care, due to several limitations. Urinary t,t-MA accounts for approximately 4% of the absorbed dose of benzene, and represents recent exposure with a half-life of 5 hours. However, t,t-MA is also produced by the metabolism of sorbic acid, which is present in many foods. Urinary SPMA is a specific biomarker of benzene and has a half-life of approximately 9 hours. SPMA represents less than 1% of benzene exposure and its production is variable due to genetic polymorphism of metabolic enzymes (IARC 2018; Hays et al. 2012; Arnold et al. 2013). CHMS reported GMs of 58–63 μg/g creatinine and 0.15–0.20 μg/g creatinine, respectively, for t,t-MA and SPMA. The 95th percentiles were 390–460 μg/g creatinine for t,t-MA and 2.7–3.1 μg/g creatinine for SPMA. A reference value for SPMA of 7.0 μg/g creatinine was determined based on 426 adult volunteers in the UK, indicating an upper margin of background exposure (Bevan 2013).
A study of gestational exposure in a region of British Columbia with significant natural gas extraction reported that whereas the median SPMA level among pregnant people was similar to that of the general Canadian population, the median t,t-MA level was approximately 3.5-fold higher (Caron-Beaudoin et al. 2018). Urinary SPMA was significantly higher in self-identifying Indigenous women compared to the general population. In urine samples collected in adults over 16 years in the Qanuilirpitaa 2017 Inuit Health Survey, GMs of urinary t,t-MA and SPMA were 187 and 1.0 μg/L, respectively. Both values were significantly higher than the CHMS GMs of 65.6 and 0.18 μg/L reported in Cycle 4. Benzene metabolites were also correlated with cotinine levels, suggesting that smoking was a key contributor to elevated benzene levels (Caron-Beaudoin et al. 2022).
Mean levels of t,t-MA among children aged 6 to 11 years of age included in the CHMS in 2009 to 2015 ranged from 78 to 81 μg/g creatinine, which is similar to means for the same age group from NHANES data for 2011 to 2012 (Health Canada 2017; Jain 2015). Research conducted in Italy demonstrated that exposure to environmental tobacco smoke and urban air pollution are key factors in children's urinary levels of benzene and metabolites. Children living in homes with people who smoke excrete significantly higher levels of urinary benzene, even if there is no smoking inside the home, or no smoking when children are present (Protano et al. 2012). Children living in urban areas in Italy were reported to have urinary t,t-MA, SPMA, and benzene levels 1.5-fold higher than those living in rural areas (Protano et al. 2010). Conversely, proximity to greenspace and vegetation has been associated with decreased urinary t,t-MA among adults in Kentucky (Yeager et al. 2020).
5.0 Health effects
This section provides a review of key health effects of benzene in humans and relevant toxicological studies in experimental animals, with supporting information from in vitro test systems. Routes of exposure other than inhalation, (in other words, ingestion and dermal), as well as mixtures including benzene (such as tobacco smoke) were excluded as they are beyond the scope of this document. Human epidemiology studies related to indoor and personal exposure to benzene were reviewed. Studies of occupational and outdoor (ambient) exposure were reviewed for additional support for effects observed in indoor environments, or to support a key effect when no relevant indoor or personal exposure studies were identified. Considering the 1 000–10 000-fold difference in occupational and environmental concentrations, exposure concentrations are reported in mg/m3 (ppm) for human occupational studies and inμg/m3 (ppb) for environmental studies. Exposure concentrations in toxicological studies are expressed in mg/m3 (ppm).
Relevant information was drawn from the following authoritative reviews of the health effects of benzene: (a) ATSDR's Toxicological Profile for Benzene (2007); (b) US EPA's Toxicological Review of Benzene (2002); (c) OEHHA's Benzene Reference Exposure Levels Technical Support Document (2014); and (d) IARC's monograph for Benzene (2018).
Additional details of the key human exposure and toxicological studies presented below are presented in Appendices B and C.
5.1 Effects in humans
There is a large body of evidence of long-term effects of benzene on humans in occupational settings, but far fewer reports on the effects of acute exposure. There is a growing body of epidemiological evidence to support the assessment of health effects at concentrations relevant to indoor residential settings.
Acute exposure to very high concentrations has been linked to CNS and other neurological effects, respiratory irritation, and hematotoxicity. Lethal doses have been reported to cause asphyxiation, respiratory arrest, CNS depression and ventricular fibrillation. However, these studies are limited by poor exposure assessment and reporting.
Epidemiological studies have found associations between benzene in indoor residential settings and impaired lung function, increased asthma prevalence, and asthma symptoms in populations including adults and children. Personal and outdoor exposure to benzene during pregnancy has been associated with preterm birth and developmental effects such as reduced head circumference and low birth weight.
Evidence of hematotoxicity—primarily depressed blood cells counts and effects on precursor cell populations in bone marrow—has been reported in many occupational studies. Altered blood cell counts have also been reported in biomonitoring studies of the general population and populations exposed to elevated levels of benzene (for example, due to environmental pollution). However, some of the effects found in the general population differ from those reported at higher (that is, occupational) exposures.
Other long-term non-cancer effects in humans include neurological effects on hearing and cognition along with effects on glucose metabolism.
Additional study details are presented in Appendix B.
5.1.1 Short-term exposure
Short-term human exposure to very high concentrations of benzene can be lethal and has been reported to cause respiratory, neurological, and hematological toxicity. However, evidence for short-term toxicity comes from a small number of reports of effects after very high occupational exposures, or environmental releases, most of which have poor exposure assessment. Two controlled human exposure studies involving benzene were identified, but they provide only limited data on health outcomes.
Acute inhalation of benzene at concentrations of 800–9 600 mg/m3 (250–3 000 ppm) caused dizziness, headache and vertigo, drowsiness, tremor, delirium, and loss of consciousness. It is estimated that exposure to 64 000 mg/m3 (20 000 ppm) for 5–10 minutes may be fatal (US EPA 2002). Lethality is attributed to asphyxiation, respiratory arrest, CNS depression and ventricular fibrillation. Autopsy results in such cases have revealed cyanosis, hemolysis, acute granular tracheitis, laryngitis, bronchitis, and massive hemorrhages and edema of the lungs and other organs (ATSDR 2007).
Respiratory effects are also reported after acute or short-term exposure in an occupational setting. Membrane irritation and shortness of breath were reported among 15 male shipyard workers exposed to over 192 mg/m3 (60 ppm) of benzene for up to 3 weeks (ATSDR 2007).
Two single controlled human exposure studies that reported health effects were identified. No adverse effects were reported in three males and three females exposed to 52–62 ppm of benzene for 4 hours (Nomiyama and Nomiyama, 1974, as cited in OEHHA 2014). In another study, human volunteers reported no subjective symptoms after exposure to up to 350 mg/m3 (110 ppm) benzene for 2 hours (Srbova 1950, as cited in OEHHA 2014). Both studies were primarily designed to assess benzene pharmacokinetics, and clinical symptoms were not investigated systematically.
One hundred and fifty-seven children were exposed to an unknown concentration of benzene for 40 days during a flaring incident at a petroleum refinery in Texas, which released over 7 000 kg of benzene along with other contaminants into the air. Exposed children experienced neurological problems (including unsteady gait, memory loss, and headaches) and upper respiratory symptoms. When the means were compared using t-tests, reduced white blood cell (WBC) counts, an increase in platelets, and increased liver enzymes (alkaline phosphatase, aspartate aminotransferase, and alanine aminotransferase) were also observed in exposed children (D'Andrea and Reddy 2014).
5.1.2 Long-term exposure (Non-cancer effects)
Respiratory toxicity
Long-term exposure to very high concentrations of benzene in an occupational setting can cause respiratory irritation. Epidemiological studies of adults and children in indoor residential settings have reported multiple respiratory effects, including reduced lung function in adults and children and increased asthma prevalence and symptoms in children. Mixed results have been found across a range of available studies. Ambient benzene exposure has been associated with reduced lung function and worsened asthma symptoms in children. However, in other studies, personal benzene exposure was not associated with lung function and cardiovascular health in asthmatic children, and indoor benzene was not associated with lower respiratory tract infection and related symptoms in infants and children.
There are few reports of respiratory effects in humans after long-term exposure to benzene in occupational settings, but few details are provided. Exposure to 106 and 189 mg/m3 (33 and 59 ppm) benzene for more than one year was found to be associated with nasal irritation and sore throat in male and female workers, respectively (ATSDR 2007).
Epidemiological studies published since 2009 have reported adverse respiratory effects associated with exposure to environmentally relevant benzene concentrations from 1 to 10 μg/m3 (0.3 to 3.1 ppb). Cakmak et al. (2014) examined the associations between residential indoor exposure to benzene and other VOCs and lung function in people who do not smoke using cross-sectional data collected in CHMS Cycle 2. A 7-day GM of 1.06 μg/m3 (0.33 ppb) was reported in the main living area of non-smoking homes. Significantly reduced lung function (FEV1/FVC) was associated with a log-transformed interquartile range (IQR) increase in benzene of 1.31 μg/m3 (0.0013 mg/m3) after sociodemographic covariate adjustments. Lung function in males was significantly reduced by 1.75% (95% confidence interval [CI], -2.71% to -0.79%), but a similar effect was not reported for females. An IQR increase in the indoor concentration of benzene was also associated with a statistically significant decline of 2.3% in FEV1/FVC in children under age 17 (95% CI, -3.7% to -0.9%) and a decline of 1.45% in people aged 39–64 (95% CI, -2.44% to -0.45%). Models were adjusted for sociodemographic covariates (age, gender, income, and education) but not for other VOCs. Benzene and nine other VOCs (decanal, 2-furancarboxaldehyde, hexanal, nonanal, octanal, styrene, a-pinene, 2-methyl-1,2-butadiene and naphthalene) were all negatively associated with lung function.
In a prospective panel study, Martins et al. (2012) evaluated the relationship between lung function and indoor and outdoor exposure to benzene and other air pollutants measured in the homes and schools of a group of children living in Portugal. When total exposure in homes (bedrooms) and schools (courtyard and classroom) was estimated, mean benzene concentrations were 2.9–10.7 μg/m3 (0.9–3.3 ppb) in winter and 1.0–1.6 μg/m3 (0.3 to 0.5 ppb) in summer. An increase of 10 μg/m3 (3.3 ppb) of benzene per week was significantly associated with a 4.33% decrease in FEV1(95% CI, -7.13% to -1.13%), a 1.17% decrease in FEV1/FVC (95% CI, -3.24% to -0.18%), a 5.89% decrease in FEF25-75%(95% CI, -10.16% to -1.62%), a 2.79% increased change in FEV 1 (DFEV1) (95% CI, 0.92%–4.65%), and decreased pH of exhaled breath condensate (an indicator of airway inflammation). Models were adjusted for parental smoking and other sociodemographic, home and physical characteristics but not for co-pollutants. An increase in total exposures to PM10, NO2, toluene and ethylbenzene were also associated with a decrease in lung function in these children.
Indoor benzene exposure has also been associated with asthma prevalence in children. In a case-control study conducted in France, the median indoor benzene concentration was 3.85 μg/m3 (1.2 ppb) in the living room of asthmatic children and 1.9 μg/m3 (0.6 ppb) in the homes of non-asthmatic children (Rive et al. 2013). Odds ratios (OR) of 8.11 for asthma (95% CI, 1.41–46.43) and 10.10 for being woken by wheezing (95% CI, 2.06–49.78) were reported for children with exposures above the sample median of 2.4 μg/m3 (0.8 ppb). Models were adjusted for exposure to passive smoking, atopy, family allergy, age, and sex, but not for co-pollutants. A meta-analysis of 15 epidemiological studies of benzene exposure in both indoor and outdoor air reported a pooled relative risk (RR) for asthma of 1.08 (95% CI, 1.02–1.14), per 1 μg/m3 increase of benzene (Liu et al. 2022). A significant association was also found for subgroups of cross-sectional studies (pooled RR = 1.07; 95% CI, 1.00–1.14), children (pooled RR = 1.09, 95% CI, 1.02–1.16), and Western countries (pooled RR = 1.08, 95% CI, 1.03–1.14). In the same study, associations between benzene and rhinitis or bronchitis were not statistically significant (rhinitis pooled RR = 1.04, 95% CI, 0.99–1.10; bronchitis pooled RR = 1.58, 95% CI, 0.80–3.09).
Personal exposure to benzene and other air pollutants were measured for 10 consecutive days in a panel study, to assess the associations between exposure to benzene and other air pollutants and changes in pulmonary function and selected indicators of cardiovascular health (including blood pressure, pulse rate and oxygen saturation) among asthmatic children living in an urban industrial area in Montreal, Quebec (Smargiassi et al. 2014). The median benzene concentration experienced by these asthmatic children was 2.10 μg/m3 (0.7 ppb). Exposure to benzene was not associated with a decline in lung function or cardiovascular effects in this group of children after adjustments for parental smoking and other confounders. Co-pollutants were not adjusted for in this study. Two cohort studies of lower respiratory tract infection in infants and symptoms such as cough and wheeze failed to find an association with indoor benzene exposure, which ranged from 1.23 to 4.3 μg/m3 (0.4–1.3 ppb) after adjustment for parental smoking but not for co-pollutants (Ferrero et al. 2017; Vanker et al. 2017).
Epidemiological studies of ambient benzene, with mean benzene exposures ranging from 0.83 to 3.0 μg/m3 (0.3–0.9 ppb), also showed positive correlations between ambient benzene exposure and reduced lung function or exacerbation of asthma symptoms in children living in Argentina, Spain, and France (Wichmann et al. 2009; Morales et al. 2015; Charpin et al. 2009; Zhou et al. 2013). All of these studies adjusted for parental smoking but not for ambient co-pollutants. Similar positive correlations were also observed for ambient exposure to other pollutants such as PM10, NO2, CO, and total VOCs.
Reproductive and developmental toxicity
A small number of studies have reported reproductive effects in female workers occupationally exposed to benzene. Epidemiology studies of reproductive effects in an indoor residential environment were not identified. However, personal and ambient exposure to benzene during pregnancy has been associated with preterm birth and developmental effects such as reduced head circumference and low birth weight. In most studies, an adjustment was made in regression models for the mother's age, physical characteristics and socioeconomic or sociodemographic factors, but not for co-pollutant exposure.
Studies published prior to the previous Health Canada Guidance (Health Canada 2013a) addressed the potential reproductive toxicity of benzene for occupational exposures to benzene at relatively high concentrations. Associations between occupational benzene exposure and spontaneous abortions, menstrual disturbances, and ovarian atrophy in exposed female workers were reported in some studies, while the findings of other studies regarding reproductive toxicity and benzene exposure were inconclusive (ATSDR 2007; US EPA 2002; Olsson et al. 2018). One study examined the reproductive competence of male workers exposed to benzene and found no statistical difference in the frequency of spontaneous abortion in partners of fathers exposed to benzene before conception compared to partners of unexposed fathers (ATSDR 2007).
There are few early studies regarding the association between benzene exposure and human developmental effects (ATSDR 2007; US EPA 2002). In one case report, a worker exposed to benzene throughout her pregnancy suffered reduced blood cell counts and increased chromosomal aberrations, but her offspring showed no evidence of chromosomal effects (US EPA 2002; ATSDR 2007).
Several non-occupational studies published since 2009 have assessed the association between maternal benzene exposure and developmental endpoints in infants. A cohort study conducted in France assessed the relationship between personal exposure to benzene and fetal growth (Slama et al. 2009). Benzene was measured for 7 days during the 27th gestational week of pregnancy by an air sampler carried by the participants. The cohort comprised non-smoking pregnant women and their median benzene exposure was 1.8 μg/m3 (0.0018 mg/m3). The authors observed non-significant dose-dependent decreases in birth weight and in head circumference at birth when mothers with benzene exposure greater than 1.4 μg/m3 (0.4 ppb) were compared with mothers with an exposure level below 1.4 μg/m3 (0.4 ppb). When benzene was analyzed as a log-transformed continuous variable, a statistically significant decrease of 68 g in mean birth weight (95% CI, -135 to -1 g) and a borderline significant decrease of 1.9 mm in head circumference (95% CI, -3.8–0.0 mm) were found. Regression models were adjusted for confounders including those related to physical measurements of the fetus and mother at week 27, maternal occupational exposure to paint and pesticides, and maternal passive smoking. Models were not adjusted for co-pollutants.
Associations between ambient benzene concentration and various developmental endpoints including term low birth weight, preterm birth, birth length, and head circumference have also been reported. A case-control study conducted in the US assessed maternal outdoor benzene exposure from traffic exhaust and the risk of low birth weight (Ghosh et al. 2012). Exposure to benzene (mean = 3.5 μg/m3, 1.1 ppb) during the third trimester was found to be associated with a borderline significant OR of 1.03 (95% CI, 1.00–1.05) per IQR (2.6 μg/m3, 0.8 ppm) increase for term low birth weight in infants. Similar associations were reported for toluene, ethylbenzene, and xylenes. Models were adjusted for gestational age, maternal age, and socioeconomic factors, but no adjustment was made for smoking or co-pollutants. In another study, which assessed outdoor benzene exposure in a multicentre cohort in Spain, no significant relationship was observed between outdoor benzene levels (mean = 1.6 μg/m3, 0.5 ppm) and birth weight, length, or head circumference. An adjustment was made for smoking and other maternal characteristics but not for co-pollutants (Estarlich et al. 2011). A meta-analysis of three epidemiological studies of indoor and outdoor exposure to benzene reported a pooled RR for low birthweight of 1.12 (95% CI: 1.05–1.19) per 1 μg/m3 increase of benzene (Liu et al. 2022).
In a case-control study of pregnant US women (mean exposure = 2.1 μg/m3, 0.7 ppb), an increased risk of preterm birth (OR=1.09, 95% CI, 1.06–1.13) per IQR (0.64 μg/m3, 0.20 ppb) increase in traffic-related outdoor benzene exposure during the entire pregnancy was observed. Results are presented for a single pollutant model adjusted for maternal age, ethnicity, education and parity. Other traffic-related exposures including PAHs, elemental carbon, and diesel were highly correlated with exposure to benzene, and were also associated with increased risks of preterm birth (Wilhelm et al. 2011). A cohort study conducted in Spain reported an increased risk of preterm birth associated with ambient benzene exposure (median = 1.3 μg/m3, 0.4 ppb) during pregnancy (Estarlich et al. 2016). After controlling for NO 2 exposure and other confounders (including smoking, sociodemographic characteristics, environmental exposures, time-activity patterns, and lifestyle variables), the authors reported an OR of 1.45 (95% CI, 1.00–2.09) per μg/m3 (0.3 ppb) increase in benzene exposure in the third trimester among pregnant women who spent more than 15 hours per day at home during their third trimester. However, a meta-analysis of two other studies failed to find a statistically significant association between benzene and preterm birth (pooled RR = 1.57; 95% CI, 0.50–4.94) (Liu et al. 2022).
Williams et al. (2019) assessed ambient exposure to benzene and other VOCs and gestational diabetes mellitus in pregnant women of four different ethnicities in a US cross-sectional study. The authors observed that a preconception benzene exposure of 1.1 μg/m3 (0.34 ppb) or higher was associated with a 25% increase in the odds (99% CI, 1.08–1.43) of developing gestational diabetes mellitus among white people and a 41% increase in the odds (99% CI, 1.12–1.77) among Asian Pacific Islanders. The authors also observed that exposure to a benzene level of 1.1 μg/m3 (0.34 ppb) or higher during the first trimester of pregnancy was associated with a 29% increase in the odds (99% CI, 1.04–1.59) of developing gestational diabetes mellitus among Asian Pacific Islanders. Similar effects were not observed among Black and Hispanic groups. Models were adjusted for maternal physical characteristics, parity, marital status and socioeconomic factors, but not for co-pollutants.
Hematotoxicity and immunotoxicity
In humans, hematotoxicity is a key adverse effect of long-term exposure to benzene in air, particularly in occupational settings. Multiple blood cell types, including erythrocytes, leukocytes, and platelets, are susceptible to benzene toxicity, which is thought to target bone marrow and early progenitor cells (ATSDR 2007; Health Canada 2013a).
At the time of the publication of the previous Health Canada Guidance (Health Canada 2013a), evidence of hematotoxicity was available only for occupational exposure to benzene, which is at least 1 000-fold higher than environmental exposure concentrations. Studies examining benzene-exposed workers revealed that chronic air exposure to benzene at concentrations of 32 mg/m3 (10 ppm) or higher was associated with various adverse hematological effects, and that severity increased with increasing levels of benzene exposure (ATSDR 2007; Health Canada 2013a). Since then, epidemiological studies have reported hematotoxicity at exposures at or below 3.2–6.4 mg/m3 (1–2 ppm) benzene (Smith 2010; Schnatter 2020).
Lan et al. (2004) reported hematological effects in workers exposed to benzene at a concentration of less than 3.2 mg/m3 (1 ppm). In this study, 250 benzene-exposed shoe workers were age- and sex-matched to 140 unexposed workers from another factory, then further divided into four exposure groups: unexposed, <3.2 mg/m3 (1 ppm), 3.2 to <32 mg/m3 (1 to <10 ppm), and ≥32 mg/m3 (10 ppm). The authors reported that total WBCs, granulocytes, lymphocytes, B cells, and platelets significantly declined with increasing benzene exposure and were lower in workers even at air exposure levels below 1 ppm, after adjusting the models for age, sex, current smoking, current alcohol drinking, BMI, recent infections, and log-transformed toluene air level when appropriate. In addition, benzene exposure decreased colony formation from myeloid progenitor cells, and progenitors were more sensitive to benzene toxicity than mature WBCs.
Recent epidemiological studies have also reported hematotoxicity at low levels of occupational exposure to benzene. Compared to unexposed workers, workers with personal exposure to <3.2 mg/m3 (1 ppm) benzene showed declines in cell types derived from myeloid progenitor cells, including granulocytes and platelets, as well as alterations in lymphoid cell types (Bassig et al. 2016). In a cross-sectional study, Harati et al. (2017) also reported hematological changes including mean corpuscular hemoglobin concentration (MCHC) and eosinophil count among workers exposed to benzene at air concentrations <3.2 mg/m3 (1 ppm). In a cross-sectional study of workers in five factories in China, significant decreases in neutrophils and mean platelet volume were reported at personal exposure to 25.0–26.2 mg/m3 benzene (7.8–8.2 ppm) (Schnatter 2010). Significantly increased odds of reduced red blood cell (RBC) counts were also observed at <1 ppm (OR 10.8, 95% CI 1.41–82.5) and at >10 ppm (16.0, 95% CI 2.11–121), although the effect was not significant at the middle dose (1 to <10 ppm).
The effect of cumulative exposure on hematotoxicity was addressed in a recent study of 317 workers from three shoe factories, stratified by age and job type, each in a separate work area with benzene concentrations ranged from 5.0 mg/m3 (1.57 ppm) to 8.3 mg/m3 (2.60 ppm), and 102 controls (Zhang et al. 2016). The exposed workers were divided into groups based on cumulative exposure, and a median value was assigned to each group: 3.55 ppm-years (11.4 mg/m3-years); 6.51 ppm-years (20.8 mg/m3-years); 10.72 ppm-years (34.3 mg/m3-years); 20.02 ppm-years (64.1 mg/m3-years); and 40.71 ppm-years (130.2 mg/m3-years). Mean WBC counts were lower in the exposed workers as a whole, compared to the unexposed workers, and statistically significant decreases were reported in the three highest exposure groups. Trend testing indicated significant dose-response for the younger, older, and age-pooled groups. Benchmark dose modelling of WBC counts for all age groups was conducted using Hill and Log-probit models, with the lower bounds ranging from 1.37 to 11.82 ppm-years (4.4–37.8 mg/m3-years).
No studies of hematotoxicity in relation to personal or indoor air exposure to benzene were identified. However, in biomonitoring studies of populations that did not have occupational exposure, blood concentrations of benzene have been associated with hematotoxic effects. It is noteworthy, though, that some reported changes are inconsistent with those seen in occupational settings. Elderly residents from a community less than 3 km from a petrochemical plant had mean blood concentrations of BTEX that were 1.2–6.7 times higher than a control group selected from a community with no nearby petrochemical industry. MCHC and platelets were inversely correlated with log-transformed blood benzene concentrations (Chen, Sun et al. 2019). Similarly, blood benzene concentrations in non-smoking residents of the Gulf States of the US who had been exposed to emissions from oil spills in the region were associated with decreased levels of hemoglobin and MCHC and increased RBCs in comparison with the general population, as measured in NHANES (Doherty et al. 2017).
In studies of NHANES and CHMS participants, increases in blood benzene concentration were associated with altered levels of peripheral blood cells. Among NHANES subjects, an increase of one standard deviation in blood benzene levels due to tobacco smoke exposure was associated with a statistically significant increase in eosinophils, hematocrit, hemoglobin, and platelets (Vaughan Watson et al. 2021). In an analysis of CHMS Cycle 3 and 4 participants, an increase in hemoglobin, WBCs, and RBCs was found to be correlated with an increase in blood benzene level equivalent to the GM (Cakmak et al. 2020). Among this population, people who smoke has a larger increase in WBC per GM-increase in blood benzene, compared to people who do not smoke.
As with hematotoxicity, immunotoxic effects have been reported in humans with occupational exposure to benzene. Earlier studies have reported that benzene alters both humoral and cellular immunity. Painters chronically exposed to benzene (10–22 mg/m3, 3–7 ppm), toluene and xylene showed altered serum immunoglobulin M, G, and A levels. In addition to reduced WBCs and other blood components (as described above), workers exposed to benzene are reported to have altered bone marrow histopathology and enlarged spleens at concentrations ranging from 3.2–3 400 mg/m3 (1–1 060 ppm) (ATSDR 2007). Available mechanistic evidence supports an immunosuppressive effect of benzene (IARC 2018). Increased immunoglobulin G (IgG) was found to be positively correlated with air benzene levels and with benzene urinary metabolite t,t-MA (Bogadi-Šare 2000; Dimitrova et al. 2005). In a recent occupational study, levels of plasma concentrations of soluble CD27 and CD30, two immune markers indicative of B-cell activation were compared in workers with and without benzene exposure (Bassig et al. 2016). In addition to significant dose-dependent decreases in total lymphocytes and myeloid cells (such as granulocytes and platelets), workers exposed to a median benzene level >3.2 mg/m3 (1 ppm) showed significant dose-dependent declines in B cells (15%), CD4+ T cells (14%) and the CD4+/CD8+ ratio (14%). Benzene exposure levels over 32 mg/m3 (10 ppm) were associated with a significant decrease in soluble CD27 and a non-significant decrease in soluble CD30 compared to unexposed workers.
Other effects
Neurotoxicity
Few studies have examined the neurological effects of benzene, although neurological effects are a well-known outcome of occupational exposure to solvents, in general. Chronic neurological effects including frequent headaches, feeling tired, sleep disturbance and memory loss have been reported by workers exposed to 20–50 mg/m3 (6–15.6 ppm) benzene and <5 mg/m3 toluene. Workers chronically exposed to benzene at levels up to 671 mg/m3 (210 ppm) who developed aplastic anemia or pre-leukemia also had adverse effects on their motor skills and reflexes pointing to effects on peripheral nerve axons (US EPA 2002; ATSDR 2007). Unfortunately, older studies of benzene-induced neurological effects are not considered fully reliable due to co-exposures and poor exposure assessment.
Studies published since the previous Health Canada Guidance (Health Canada 2013a) have also reported neurological effects in workers exposed to benzene and benzene-based solvents. A higher risk of poor cognitive performance (OR=1.58) among workers with high exposure to solvents containing benzene compared to unexposed workers was reported in a large French occupational cohort (Berr et al. 2010). Another study examined cognitive deficits in retirees from the same cohort and concluded that a high level of lifetime occupational exposure to benzene was significantly associated with poor cognition (Sabbath et al. 2014). In addition, Sabbath et al. found that solvent exposure rates were higher among workers with less than secondary school education, and that among less educated workers, there was a dose-response relationship between lifetime benzene exposure and risk of poor cognition (Sabbath et al. 2012). No dose-response relationship was found in workers with higher levels of education. In these studies, exposure was defined in a semi-quantitative manner based on self-reporting and job-exposure matrices, and the extent of co-exposure is not clearly documented.
No studies were identified that investigated associations between residential indoor air concentrations and neurological effects. Associations between environmental benzene exposure and neurological outcomes have been reported in a small number of biomonitoring studies involving children, adolescents, and adults. In a Belgian study, the authors reported a link between a decline in sustained attention in adolescents and increased levels of traffic exposure (estimated from urinary t,t-MA and survey responses about traffic exposure) and with urinary t,t-MA alone (Kicinski et al. 2016). In South Korean children, urinary t,t-MA was significantly higher in children with otitis media effusion than in controls (Kim et al. 2017). Staudt et al. found a statistically significant association between blood benzene concentrations and increased adjusted odds of high-frequency hearing loss among US adults (Staudt et al. 2019).
Glucose metabolism and oxidative stress
Long-term environmental exposure to benzene has been implicated in glucose metabolism and insulin resistance in human studies. A significant association between urinary t,t-MA, and insulin resistance, fasting blood glucose and fasting blood insulin was reported in children and adolescents in Iran (Amin et al. 2018). Significant increases in superoxide dismutase and malondialdehdye (markers of oxidative stress) were also reported in subjects with intermediate levels of t,t-MA compared to those with low levels. In another study, levels of urinary t,t-MA showed a dose-dependent association with insulin resistance (as measured by the HOMA-IR) and urinary malondialdehdye in adults over 60 years of age (Choi et al. 2014).
5.2 Toxicological studies
Studies of the effects of inhalation exposure to benzene in toxicological studies support the effects seen in humans with regard to some acute effects, reproductive and developmental effects, and hematological effects. Respiratory effects are reported in a small number of short-term animal studies, and no studies were identified on of neurological effects of long-term benzene exposure.
Benzene has been reported to cause severe acute toxicity to animals at very high exposure concentrations (>16 000 mg/m3). Single acute inhalation exposures to benzene in this range were reported to cause increased respiratory rates, CNS effects and death in animal studies. Short-term inhalation exposures have also been reported to cause hematotoxic and immunotoxic effects, as well as neurobehavioural effects such as dizziness, headache, vertigo, drowsiness, tremor, delirium, loss of consciousness and hearing impairment. Short-term exposure has also been reported to cause apoptosis in lung tissue.
Gestational exposure to inhaled benzene was reported to cause reduced fetal body and organ weights, bone marrow toxicity and hematotoxic effects in offspring. Hematotoxic and immunotoxic effects in adult animals include decreased spleen weight, decreases in circulating blood cells, bone marrow damage and depressed immune response.
Additional study details are presented in Appendix C.
5.2.1 Short-term exposure
Benzene has been shown to cause severe acute toxicity in experimental animals at concentrations greater than 16 000 mg/m3 (5 000 ppm) (Health Canada 2013a; OEHHA 2014). Acute inhalation exposure to single, high doses of benzene in the range of 32 000 mg/m3 (10 000 ppm) resulted in CNS effects and death in experimental animals (Health Canada 2013a). LC 50 values (50% lethal concentration) in mice and rats ranged from 31 400 mg/m3 to 43 800 mg/m3 (9 800 ppm–13 700 ppm). Increased respiratory rates occur in mice within 5 minutes of the onset of exposure to a dose of 18 800 mg/m3 (5 800 ppm) (OEHHA 2014).
Inhalation of 960 mg/m3 (300 ppm) benzene for 7 days caused an increase in apoptotic changes in the parenchymal lung tissues of rats. Increases of 55%–73.5% in the apoptotic index were reported for bronchiolar, terminal bronchiolar and alveolar epithelia (Weaver 2007). Neurobehavioral effects were observed in mice after a range of short-term exposures (2.5–960 mg/m3; 0.8–300 ppm). These included CNS effects such as lethargy and behavioural effects including reduced milk licking, grip strength, locomotor activity, and maze performance (OEHHA 2014; US EPA 2002).
Hematotoxicity and immunotoxicity are apparent in animal studies with short-term inhalation exposure. Effects in mice due to inhalation exposure to 96 mg/m3–320 mg/m3 (30–100 ppm), for 6 hours/day over 5–7 days, include decreased spleen and bone marrow cellularity, decreased spleen weight, decreased resistance to bacterial infection and reduced T- and B-lymphocytes in the spleen. In a study used to develop reference exposure levels for short-term exposure guidelines developed by the Agency for Toxic Substances and Disease Registry (ATSDR 2007), Texas Commission on Environmental Quality (TCEQ 2015) and L'Agence Nationale de Sécurité Sanitaire de l'Alimentation, l'Environnement at du Travail (ANSES 2008), male mice were exposed to 0, 32, 96, 320 or 960 mg/m3 (0, 10, 30, 100 or 300 ppm) benzene, 6 hours/day for 6 days. Circulating lymphocyte counts and femoral bone marrow B-lymphocyte colony-forming ability were reduced at all levels of exposure. Erythrocyte counts, femoral B-lymphocyte and splenic B-lymphocytes were significantly reduced at levels of 320 and 960mg/m3; splenic B-lymphocyte colony-forming ability was reduced at 960 mg/m3 (Rozen et al. 1984).
More recent studies shed light on the molecular pathways implicated in hematotoxicity due to benzene exposure. Male mice, either wild type or carrying a hepatocyte-specific homozygous deletion of the Ppp2r1a gene (encoding the protein phosphatase 2 a subunit) were exposed to benzene concentrations of 0, 3.2, 32 or 320 mg/m3 (0, 1, 10 or 100 ppm), 6 hours/day, 6 days/week for 28 days. Significant decreases in peripheral lymphocytes were observed at all doses in wild type mice; decreases in all measured blood parameters (RBCs, WBCs, platelets, lymphocytes and mean corpuscular volume) were noted at the highest dose. In the homozygous mice, significant decreases in lymphocytes were only observed in the 100-ppm group and reductions in RBCs, WBCs and mean corpuscular volume were less profound than in wild type mice. Homozygous mice also produced lower levels of urinary SPMA and exhaled benzene metabolites than wild type mice, suggesting that protein phosphatase 2 has a protective effect on benzene hematotoxicity and plays a role in the regulation of benzene metabolism (Chen, Guo et al. 2019).
Histone deacetylase was also implicated in benzene hematotoxicity. Male mice were injected intraperitoneally with histone deacetylase inhibitors (trichostatin A or MCP30) prior to exposure to 0 or 960 mg/m3 (300 ppm) benzene, 6 hours/day, 5 days/week for 8 weeks. Treatment with benzene alone resulted in a significant decrease in peripheral blood cells counts (hemoglobin, WBCs, and platelets) and a decrease in hematopoietic cells in bone marrow. However, mice treated with histone deacetylase inhibitors showed statistically significantly less severe changes in these parameters. The expression and activity of Topoisomerase IIa, a target of histone deacetylase, was also increased in mice treated with benzene and trichostatin A or MCP30 compared to benzene alone (Chen et al. 2016).
Acute and short-term exposure to benzene in animals caused effects on hearing, and the cardiovascular system. Anesthetized and tracheotomized rats exposed to 9 600 mg/m3 (3 000 ppm) benzene for two 15-minute intervals, separated by 20 minutes of fresh air, had significant increases in middle-ear reflex amplitude changes, without effects on cochlear histopathology (Wathier et al. 2019). Cardiovascular disease-related effects were observed in male mice exposed to 0 or 50 ppm (160 mg/m3) benzene, 6 hours/day for 6 weeks. Circulating angiogenic cells (Flk+/Sca+) were statistically significantly reduced by 48% in mice exposed to benzene, compared to mice exposed to filtered air. Dyslipidemia was induced in benzene-exposed mice, which showed increased total cholesterol, plasma low-density lipoprotein (LDL), and plasma high-density lipoprotein (HDL) relative to control animals. As a result, a 23% increase in the LDL:HDL ratio was reported for benzene-exposed mice (Abplanalp et al. 2017).
5.2.2 Long-term exposure
Respiratory toxicity
Few studies were identified that address respiratory effects following long-term exposure to benzene in animals. No effects were found in lung tissue of mice and rats exposed to 0, 320, or 960 mg/m3 (0, 100, or 300 ppm) of benzene for 5 days/week, 6 hours/day during their lifetime. In addition, adverse histopathological effects were not observed in lung tissue in mice exposed to 960 mg/m3 (300 ppm) benzene for life (ATSDR 2007).
Reproductive and developmental toxicity
Embryotoxic and fetotoxic effects, such as reduced fetal body and organ weights, have been reported in animal studies with maternal inhalation exposure concentrations as low as 150 mg/m3 (47 ppm) during gestation (Health Canada 2013a; OEHHA 2014). Minor skeletal effects were reported at much higher concentrations. In mice, in utero exposure in the range of 16–64 mg/m3 (5–20 ppm) resulted in significant bone marrow toxicity and hematopoietic effects, such as suppression of erythropoeitic precursor cells, a decrease in early nucleated red cells (basophilic normoblasts) and enhanced granulopoiesis (OEHHA 2014). Testicular degeneration was observed in rabbits and guinea pigs exposed to benzene concentrations of 80–88 ppm (261–277 mg/m3) for 8 months (Health Canada 2013a, OEHHA 2014).
Hematotoxic effects, including a decrease in circulating and late erythroid precursor cells and an increase in hepatic hematopoietic blast cells and non-dividing granulopoietic precursor cells, were observed at post-natal day 2 in offspring of pregnant female mice exposed to 64 mg/m3 (20 ppm) for 6 hours per day, from gestational day 6 to 16. They exhibited a similar pattern six weeks after birth (Keller and Snyder 1988).
Hematotoxicity and immunotoxicity
Hematotoxicity and immunotoxicity were observed following subchronic and chronic exposure of experimental animals to benzene at a wide range of concentrations (<3.2–9600 mg/m3 [<1–3 000 ppm]) (Health Canada 2013a). Impaired hematopoietic function appears to be the more sensitive effect, as evidenced by changes in circulating blood cells (leukopenia, erythrocytopenia, lymphocytopenia, thrombocytopenia, pancytopenia and aplastic anemia), bone marrow damage and depressed immune response (Health Canada 2013a). Mice are considered more sensitive than rats or rabbits to benzene-related hematological effects; in mice, effects at 32 mg/m3 (10 ppm) include decreased spleen weight and decreases in circulating leukocytes, as well as decreases in peripheral lymphocytes, RBCs, and colony-forming units in the bone marrow and spleen (Health Canada 2013a; OEHHA 2014).
Other effects
Neurotoxicity
Although several animal neurotoxicity studies were found that deal with short-term exposure to benzene (discussed in Section 5.2.1), no studies involving long-term exposure were identified.
Glucose metabolism and oxidative stress
A 13% increase in fasting plasma glucose, a 39% increase in fasting plasma insulin, and increased homeostatic model assessment insulin resistance (HOMA-IR) scores were observed in mice exposed to 160 mg/m3 (50 ppm) benzene for 2 or 6 weeks compared to mice exposed to filtered air. Exposed mice also showed signs of insulin-stimulated Akt-phosphorylation, Nf-kb phosphorylation, and oxidative stress of liver and skeletal muscle. Treatment with a superoxide dismutase mimetic (TEMPOL) reversed benzene-induced effects on oxidative-stress, Nf-kb phosphorylation and systemic glucose intolerance (Abplanalp et al. 2019).
5.3 Genotoxicity
Benzene has been found to be genotoxic in animal and human studies, inducing micronuclei (MN), chromosomal aberrations (CA), sister chromatid exchanges (SCE) and DNA strand breaks. In animal studies, benzene exposure induces genotoxic effects in leukocytes, erythrocytes and bone marrow cells. Meta-analyses of occupational studies showed that an increased exposure to benzene is associated with increased genotoxicity. Effects including DNA adducts, strand breaks, reduced repair capacity, altered telomere length, chromosomal loss, oxidative stress and associated DNA damage are also associated with benzene exposure in humans.
5.3.1 Effects in humans
In 2018, IARC concluded that there is strong mechanistic evidence in humans that benzene is genotoxic. Genotoxicity studies of benzene in humans often assess CA or MN as markers of DNA damage in occupational settings. In cohort studies, both genotoxicity markers have been shown to be predictors of future cancer risk. Scholten et al. (2020) conducted a systematic review and meta-analysis to estimate the exposure-response relationships between occupational benzene exposure and its cytogenetic effects on CA and MN respectively (Scholten et al. 2020). Each benzene exposure increase of 3.2 mg/m3 (1 ppm) was associated with a significant increase of 0.27% CA (95% CI, 0.08%–0.47%) and a suggestive increase of 0.27% MN (95% CI, -0.23%–0.76%). The authors indicated that the results should be interpreted with caution because strong between-study heterogeneity (I 2 >90%) and evidence of publication bias were observed for both endpoints. Angelini et al. (2016) conducted a meta-analysis to examine the effect of benzene exposure on genetic damage, which was quantified using the lymphocyte cytokinesis-block MN assay on workers who had been occupationally exposed to petroleum and its derivatives. A statistically significant summary mean difference of 1.64 in MN frequency (95% CI, 0.80–2.47) in peripheral blood lymphocytes was found for workers exposed to benzene or BTX compared to unexposed workers (Angelini et al. 2016).
Two studies reported higher levels of DNA adducts in blood cells and nasal epithelia of children or adults living in urban or industrial areas who had higher levels of benzene exposure compared to those in rural areas (Ayi-Fanou et al. 2011; Peluso et al. 2013). Greater DNA damage, as indicated by % tail DNA, or increased signs of DNA strand breaks and reduced DNA repair capacity were reported in two occupational studies comparing workers exposed to benzene versus the unexposed (Li et al. 2017; Ruchirawat et al. 2010). Chromosomal loss associated with benzene exposure was also reported in several occupational studies (Bassig et al. 2016; Ji et al. 2012; Zhang et al. 2012).
Benzene exposure has also been associated with altered telomere length. While Hoxha et al. (2009) found reduced relative telomere length in workers exposed to traffic-related benzene exposure, Bassig et al. (2014) found increased telomere length in workers exposed to >31 ppm benzene.
IARC also concluded that there is strong evidence from occupational studies indicating that benzene may induce oxidative stress and associated oxidative DNA damage, such as decreased serum glutathione levels, increased lipid peroxidation, increased reactive oxygen species, oxidative protein damage, and/or decreased antioxidant capacity (IARC 2018). In one study, significant correlations were reported between benzene exposure and changes in glutathione-S-transferase, superoxide dismutase, lipid peroxidation, and reactive oxygen species in gasoline filling station workers compared with workers not exposed to benzene (Uzma et al. 2010). Another study reported increased levels of blood mitochondrial DNA copy number in occupationally exposed individuals relative to unexposed referents and suggested that this increase reflects mitochondrial DNA damage and dysfunction (Carugno et al. 2012).
5.3.2 Toxicological studies
In vivo and in vitro animal studies have shown that benzene is genotoxic, inducing DNA damage and chromosomal changes (Health Canada 2013a; OEHHA 2014; IARC 2018). In bacterial cells, benzene and its metabolites have clastogenic and aneugenic effects, producing MN, CA, SCE and double-strand breaks. In isolated animal cells, benzene has demonstrated the ability to inhibit DNA and RNA synthesis. In vivo, benzene induces CA in lymphocytes (mice) and bone marrow cells (rats and hamsters) and increases the incidence of MN in lymphocytes (rat), bone marrow (mice and hamsters) and peripheral erythrocytes (mice). SCE were observed in rat and mouse lymphocytes, as well as mouse fetus, liver and bone marrow. Gene mutations and polyploidy were observed in mouse lymphocytes (Health Canada 2013a; OEHHA 2014). Benzene also induces DNA adducts in bone marrow and leukocytes of animals exposed in vivo. Benzene metabolites, especially hydroquinone and 1,4-benzoquinone, directly inhibited topoisomerase II in exposed mice (IARC 2018).
Two studies published since the previous Health Canada Guidance (Health Canada 2013a) address mechanistic aspects of benzene genotoxicity. Exposure of diversity-outbred mice (in other words, genetically diverse mice) to up to 320 mg/m3 (100 ppm) benzene, for 28 days resulted in substantial increases of 400%–570% MN in bone marrow, reticulocytes and erythrocytes. A benchmark benzene concentration of 0.656 mg/m3 (0.205 ppm) was calculated for benzene-induced chromosomal damage. Overexpression of sulfotransferases were inversely correlated with genotoxicity, suggesting a protective role for sulfating benzene metabolites (French et al. 2015).
Mice exposed to 160 mg/m3 (50 ppm) benzene, either continuously for 8 days or intermittently for 8 days spread over 15 days, had significantly increased MN frequency in bone marrow. Continuous exposure led to considerably higher MN frequency than intermittent exposure. Co-exposure of benzene with toluene also significantly increased MN frequency, compared to benzene alone. Depletion of glutathione decreased MN frequency, implicating glutathione or a benzene glutathione conjugate in benzene-induced genotoxicity (Bird et al. 2010).
5.4 Carcinogenicity
Benzene is classified as a human carcinogen by Health Canada (Environment Canada and Health and Welfare Canada 1993), the US EPA (US EPA 2002) and IARC (IARC 1987, 2018).
IARC concluded that there is sufficient evidence demonstrating that benzene causes acute myeloid leukemia (AML) and acute non-lymphocytic leukemia (ANLL) in human adults, and data support a positive association between benzene exposure and AML in children. Varying levels of epidemiological evidence lend support for positive associations between benzene exposure and non-Hodgkin lymphoma (NHL), chronic lymphoid leukemia, multiple myeloma, chronic myeloid leukemia (CML), and lung cancer in adults (IARC 2018; ATSDR 2007). A minority of the IARC working group members concluded that benzene also causes NHL (IARC 2018).
Almost all of the studies demonstrating the carcinogenicity of benzene in adults are from occupational cohorts, or occupationally exposed individuals in population-based studies. Evidence for the role of benzene in childhood leukemia is largely based on epidemiological studies using benzene as a proxy for traffic exposure. Studies investigating carcinogenic effects of benzene exposure in a residential indoor setting were not identified.
5.4.1 Effects in humans
Leukemia
Acute myeloid leukemia / acute non-lymphocytic leukemia
The initial classification of benzene as a human carcinogen by IARC and regulatory organizations drew on evidence from studies of occupational benzene exposure and risk of leukemia in adults, specifically AML and ANLL. Early publications from several occupational cohort studies, including a US study of rubber hydrochloride manufacturing (Pliofilm) workers, a Chinese cohort study of benzene-exposed workers, and an Australian study of petroleum workers, consistently demonstrated higher risks of AML and ANLL in benzene-exposed workers (Rinsky et al. 1981, 2002; Yin et al. 1987, 1996; Glass et al. 2003, 2005). Exposures were highest in the Pliofilm and Chinese cohorts, with average exposure from <10 to >1 000 mg/m3 (<3 to >310 ppm) and cumulative exposure ranging from <10 to >400 ppm-years (<32 to >1 280 mg/m3-years) (Rinsky et al. 1981, 2002; Hayes et al. 1996). Cumulative exposures in the Australian cohort were much lower, from 0.005 to 57.3 ppm-years (0.016–183.4 mg/m3-years) (Glass et al. 2003, 2005).
In recent years, new data from occupational cohorts and general-population studies, with improved assessment of benzene exposure, provided additional evidence to support the association between exposure to benzene and development of AML/ANLL. Moreover, there is growing evidence demonstrating exposure-response trends between quantitative occupational benzene exposure data and AML/ANLL risk. In a pooled analysis of three updated nested case-control studies of petroleum workers from Canada, the United Kingdom, and Australia, the authors reported a relatively low level of exposure to benzene in comparison with other occupational studies (Schnatter et al. 2012; Rushton et al. 2014). The estimated mean benzene exposure concentration was 0.62–0.96 mg/m3 (0.2–0.3 ppm) in this study and the median cumulative benzene exposure for AML cases was 1.2 ppm-years (3.84 mg/m3-years). Elevated ORs were observed for cumulative, average, maximum, duration, peak, and dermal exposure to benzene and AML risk, although none were statistically significant. A similar trend of elevated hazard ratios with relatively low cumulative benzene exposure (0.124–0.948 ppm-years (0.397–3.03 mg/m3-years)) was reported in a case-cohort analysis of Norwegian offshore oil industry workers; however, the results were not statistically significant (Stenehjem et al. 2015).
Two population-based studies also investigated AML risk in relation to occupational benzene exposure. In a case-control study of patients diagnosed with AML in Shanghai hospitals, the authors observed that exposure to benzene was associated with a significant increase in risk of AML (OR, 1.43; 95% CI, 1.05–1.93). Moreover, they found a significant monotonic exposure-response relationship between maximum occupational benzene exposure and AML risk. Benzene exposure was self-reported by patients in interviews about occupational history. Categorical analysis also showed a significantly increased OR of 2.05 (95% CI, 1.05–3.98) when exposed patients with more than 3.1 ppm (10 mg/m3) of maximum exposure were compared to patients that were not occupationally exposed to benzene (Wong et al. 2010). A population-based nested case-control study in four Nordic countries estimated benzene exposure using occupational information obtained from census records and found no association between exposure to solvents containing benzene and AML risk (Talibov et al. 2014).
IARC conducted meta-analyses on the association between occupational benzene exposure and the risk of AML using data from 13 studies. A statistically significant meta-OR of 1.54 (95% CI, 1.16–2.05) was reported for occupational benzene exposure and the odds of AML incidence or mortality. Further meta-regression analyses were carried out using data from six occupational cohort studies with quantitative exposure assessment to investigate the exposure-response trend between cumulative benzene exposure and AML. The results strongly support a linear exposure-response relationship for AML and cumulative benzene exposure (IARC 2018).
A study on AML incidence and mortality in Canada from 1992 to 2010 at various levels (province/territory, city, and postal code) revealed AML clusters in Sarnia and other industrial cities in Ontario near the U.S. border, including Sault Ste. Marie, Thunder Bay, St. Catharines, and Hamilton (Ghazawi et al. 2019). It was found that Sarnia, a city with the highest concentration of petrochemical plants in Canada (over 60 refineries and chemical plants), had 106.81 (95% CI, 70.96–161.86) AML cases per million individuals per year, a rate over 3 times higher than the national average. Although the findings did not associate individual-level benzene exposure with AML in these cities given the ecological study design, the study raised the possibility that pollution from local petrochemical plants, a known driver for ambient benzene, may be implicated as a risk factor for AML in Sarnia, Ontario. Future studies with better benzene exposure assessment at the individual level are required to confirm this association.
Chronic myeloid leukemia, myeloproliferative disorder, and myelodysplastic syndromes
Several occupational studies have assessed occupational benzene exposure and CML, myeloproliferative disorder (MPD) or myelodysplastic syndromes (MDS) in workers from various countries.
Two studies examined workers' risks of CML, MPD, and MDS associated with relatively low concentrations of benzene exposure, using data from the same pooled case-control studies nested within three occupational cohorts from Canada, Australia, and the United Kingdom described in the section above (Schnatter et al. 2012; Glass et al. 2014). Average exposure levels reported in these studies were found to be much lower than in other occupational studies of benzene. The mean exposure was 0.3 ppm (0.96 mg/m3) for CML cases, 0.17 ppm (0.54 mg/m3) for MPD cases, and 0.2–0.3 ppm (0.64–0.96 mg/m3) for MDS cases. A consistent monotonic trend was reported between cumulative benzene exposure and MDS. The ORs for MDS were 4.33 (95% CI, 1.31–14.3) when workers with the highest tertile of exposure were compared with those with the lowest tertile of exposure (>2.93 vs. ≤ 0.348 ppm-years; >9.38 vs. ≤ 1.11 mg/m3-years), and 6.32 (95% CI, 1.32–30.2) when workers with peak exposures over 3 ppm (9.6 mg/m3) were compared with workers with no peak exposure. The evidence for CML and MPD was less clear. Although some ORs were elevated for higher tertiles of benzene exposure when compared to the lowest tertile, overall, increases in risk of CML or MPD associated with benzene exposure were not statistically significant.
Non-Hodgkin lymphoma
NHL is a heterogeneous classification of lymphoma including chronic lymphocytic leukemia, multiple myeloma, and acute lymphocytic leukemia, follicular lymphoma, mantle cell lymphoma, diffuse large B-cell lymphoma, and hairy cell leukemia. The definition of NHL has changed over time due to changes in disease classification, and different epidemiological studies have examined different types of NHL. The evidence for potential carcinogenicity for NHL associated with benzene exposure is not clear and this is likely complicated by the etiological heterogeneity of the disease classification. A small number of occupational studies have reported associations between benzene exposure and risk of NHL (Koh et al. 2011, 2014; Collins et al. 2015; Linet et al. 2015). Although some studies showed positive associations, none of the findings were statistically significant.
Lung cancer
There is limited evidence on the potential carcinogenicity for lung
cancer associated with benzene exposure. IARC concluded that there was
a positive association for benzene and cancer of the lungs, although a
small minority of working group members thought that a positive
association was not observed (IARC 2018). A Canadian case-control study
found an association between long-term ambient exposure to benzene and
other air pollutants and increased lung cancer risk in Toronto
residents (Villeneuve et al. 2014). The estimated benzene concentration
was 0.65 μg/m3 (0.2 ppb) in lung cancer cases. The authors
observed that an IQR (0.15 μg/m3; 0.05 ppb) increase in
time-weighted average benzene exposure across past residences was
associated with an OR of 1.84 (95% CI, 1.26–2.68) after model
adjustment for age, sex, smoking, second-hand smoke, and other
confounders. Significant relationships were also reported for NO 2 and total hydrocarbons in this study.
Childhood leukemia
Several studies were identified that examined the association between ambient benzene exposure (as a proxy of traffic exposure) and childhood hematopoietic cancer risks. Most studies focused on childhood leukemia (the most common type of childhood cancer) and estimated maternal exposure to ambient benzene before or during pregnancy, or early-life exposure based on residential addresses. A number of studies assessed children's benzene exposure through indicators of exposure to air pollutant mixtures, such as residential proximity to traffic, gas stations and automotive repair garages, or through parental occupational exposure to solvents containing benzene. No studies evaluating childhood cancer risk associated with measured indoor exposure to benzene were identified.
Three meta-analyses examined the association between benzene and childhood leukemia (Filippini et al. 2019; Gong et al. 2019; Filippini et al. 2015). The pooled risk estimates for childhood leukemia ranged from 0.94 to 1.64. Eight of the eleven associations were positive for benzene, and six of these were statistically significant or borderline significant. A higher pooled risk estimate for benzene exposure was observed in preschoolers compared to school-aged children (Filippini et al. 2019). Two of the meta-analyses explored the exposure-response relationship between traffic-related benzene exposure and childhood leukemia (Filippini et al. 2019; Gong et al. 2019) and reported conflicting results. While Filippini et al. (2019) found no minimal threshold of benzene exposure for an increase in risk in childhood leukemia, Gong et al. (2019) did not observe an increase in risk associated with an increase in benzene exposure level.
Results from other individual case-control studies also lend support for an increased risk of childhood AML associated with outdoor benzene exposure. One case-control study reported an OR of 5.46 (95% CI, 1.12–26.51) for childhood AML associated with an increase of 1 μg/m3 (0.3 ppb) in average outdoor benzene exposure after adjusting for PM 10 exposure among a group of Italian children below 5 years of age (average estimated benzene levels ranged from <0.10 to >0.50 μg/m3; <0.033 to >0.16 ppb) (Vinceti et al. 2012). Another case-control study, this one conducted in the US, modelled children's outdoor benzene exposure using birth residence and derived average benzene concentrations ranging from 0.11 to 2.03 μg/m3 (0.034–0.63 ppb). A higher risk of AML was observed with higher benzene exposure among children born between 2005 and 2010, although the results were not statistically significant (Janitz et al. 2017).
5.4.2 Toxicological studies
IARC has concluded that there is sufficient evidence of the carcinogenicity of benzene in experimental animals (IARC 2018). The leukemogenic potential of inhalation exposure to benzene has been demonstrated in chronic toxicological studies of mice and rats. In several studies of mice, benzene inhalation of 320–960 mg/m3 (100–300 ppm), for 10 weeks to lifetime exposure, resulted in thymic lymphoma, myelogenous leukemia, hematopoietic and lymphatic neoplasms, and carcinomas of the Zymbal gland, ovaries and lungs. Significant increases in carcinoma of the Zymbal gland in female offspring and in carcinomas of the oral cavity, liver hepatomas, tumours of the haematopoietic and lymphoid tissues were observed in rats exposed in utero and postnatally to benzene levels of 640–960 mg/m3 (200–300 ppm) for a total of 104 weeks. Exposure-related increases in thymic and non-thymic lymphomas and myeloid leukemia were observed in genetically modified mice lacking p53, suggesting that the DNA repair function may be implicated in leukemogenicity (IARC 2018).
5.5 Summary of health effects and mode of action
5.5.1 Summary of health effects
Reports of health effects from acute or short-term inhalation exposure to benzene in humans relate primarily to occupational exposure or exposure to an accidental environmental release. In humans, CNS effects occur after acute inhalation of benzene at concentrations typical of high occupational exposure (800–9 600 mg/m3; 250–3 000 ppm). Respiratory irritation and shortness of breath were also reported after short-term occupational exposure. Children exposed to unknown concentrations of benzene and other contaminants during an environmental release experienced neurological effects (such as headache and memory loss), upper respiratory symptoms, and hematotoxic and hepatotoxic effects. The exposure concentrations and durations in these studies were poorly defined and may have included exposure to multiple chemicals.
Single, acute inhalation exposure to very high concentrations of benzene (>19 000 mg/m3, 5 900 ppm) causes increased respiratory rates, CNS effects and death in animals. Hematotoxicity and immunotoxic effects, such as decreased blood and immune cell counts, spleen cellularity and reduced immunity to infection result from short-term inhalation exposures ranging from 32 to 960 mg/m3 (10–300 ppm). Neurobehavioural effects, such as dizziness, headache and vertigo, drowsiness, tremor, delirium, loss of consciousness and hearing impairment, have also been observed, sometimes at very high exposure concentrations.
Recent human epidemiological studies of benzene exposure in indoor settings reported associations with lung effects, increased asthma prevalence and asthma symptoms at concentrations ranging from 1.06 to 10.7 μg/m3 (0.33–3.3 ppb), in general populations as well as in children. However, other indoor studies involving similar exposure concentrations did not find an association between benzene concentration and pulmonary function or cardiovascular effects in children, or lower respiratory tract infection in infants. Respiratory effects were observed in occupational settings after 3 weeks of exposure to over 192 mg/m3 (60 ppm), and upper respiratory tract irritation was reported after long-term occupational exposure to benzene levels of 106–189 mg/m3 (33–59 ppm). Fatal inhalation exposures result in severe effects to respiratory and other organs, including hemorrhage, and edema. Few animal studies that deal with respiratory toxicity were identified. No effects on lung tissue of mice and rats after lifetime benzene inhalation exposure of up to 960 mg/m3 (300 ppm) were reported. In a more recent study, apoptotic effects were reported in the lung tissue of rats exposed to 960 mg/m3 (300 ppm) for 7 days.
Human epidemiological studies have shown associations between gestational personal or outdoor air exposure to benzene and developmental effects. However, no indoor air studies were identified. Reduced birth weight and smaller head circumference were linked to personal exposure to benzene. Effects including low birth weight, preterm birth, low birth length, and small head circumference were reported for maternal ambient exposure to benzene concentrations ranging from 1.3 to 3.5 μg/m3 (0.4–1.1 ppb). A limited number of reports link occupational benzene exposure to spontaneous abortions, menstrual disturbances, and ovarian atrophy. Gestational exposure of rodents (inhalation) to benzene concentrations of 16–150 mg/m3 (5–47 ppm) resulted in reduced fetal body and organ weights, bone marrow toxicity and hematotoxic effects.
Hematotoxicity and immunotoxicity are well-established effects of occupational benzene exposure and they are the key effects that were used for long-term non-cancer inhalation reference exposure levels derived by the US EPA (2002), OEHHA (2014), ATSDR (2007), TCEQ (2015), and ANSES (2008) (See Appendix D, Section D2 for details). Many occupational exposure studies have reported decreases in RBCs, WBCs, and platelets, as well as effects on precursor cell types and colony-forming units in bone marrow, at benzene concentrations of 3.2 μg/m3 (1 ppm) and higher. A recent occupational study examined cumulative exposure to benzene derived lower bound benchmark concentrations (BMCLs) ranging from 4.4 to 37.8 mg/m3-years (1.37–11.82 ppm-years) for hematotoxic effects. Hematotoxic effects associated with benzene exposure have also been reported for the general population, but some of the effects are inconsistent with those seen at higher occupational concentrations. Among the CHMS and NHANES cohorts, increased blood benzene was associated with increases in hemoglobin, WBCs, RBCs and platelets. In the case of populations exposed to increased benzene levels due to pollution, increased blood benzene levels have been associated with a reduction in hemoglobin, MCHC, and platelets and an increase in RBCs.
Both environmental and occupational exposures to benzene have been associated with neurological effects in humans. Environmental exposure to benzene (as measured by urinary t,t-MA concentration) is associated with poor sustained attention in adolescents and hearing-related deficits in children and adults. Workers report CNS depression following short-term occupational exposures to >800 mg/m3 (250 ppm) benzene. Older studies of chronic occupational exposure also reported CNS depression, as well as motor and reflex effects, but the results may be unreliable due to flaws in design and exposure assessment. More recent studies have reported that long-term occupational exposure is associated with measures of poor cognition. In animal studies, short-term exposures to benzene concentrations ranging from 2.5–960.0 mg/m3 have been reported to cause CNS and behavioural effects.
In animal and human studies, benzene has been found to be genotoxic, inducing MN, CA, SCE and double-strand breaks. Meta-analyses of occupational studies have showed that increased exposure to benzene is associated with increased CA and MN frequency. Effects including DNA adducts, strand breaks, reduced repair capacity, altered telomere length, chromosomal loss, oxidative stress and associated DNA damage are also associated with benzene exposure in humans. In animal studies, benzene exposure induces CA, DNA adducts, and MN in leukocytes, erythrocytes and bone marrow cells.
Benzene is classified as a human carcinogen by Health Canada, the US EPA and IARC, and is included on Schedule 1 of the Canadian Environmental Protection Act. IARC concluded that there is sufficient evidence that benzene causes AML and ANLL in adults, and that the data support a positive association between benzene exposure and AML in children. There are varying levels of support for positive associations between benzene exposure and NHL, chronic lymphoid leukemia, multiple myeloma, CML, and lung cancer in adults. IARC has also concluded that there is sufficient evidence in experimental animals of the carcinogenicity of benzene (IARC 2018).
Key support for the leukemogenicity of benzene in humans comes from three key occupational cohorts: Pliofilm and Chinese and Australian cohorts. Meta-analyses of occupational cohort studies provide evidence for an increased risk of AML incidence or mortality with occupational benzene exposure, and a linear exposure-response relationship for AML and cumulative benzene exposure. However, recent occupational studies indicate that risk of developing AML may be associated with lower exposure levels. In a Canadian-UK-Australian combined cohort, odds of MDS were significantly increased with increasing cumulative and peak exposures. A statistically significant association between increases in outdoor benzene concentrations and lung cancer incidence was reported in a study of Toronto residents. Traffic-related benzene exposure has been positively associated with childhood leukemia. Pooled risk estimates from meta-analyses range from 0.94 to 1.64, with higher risk for preschoolers compared to school-aged children. Individual studies have reported higher odds of developing AML among children exposed to mean outdoor concentrations of 0.11–2.03 μg/m3.
5.5.2 Mode of action
The carcinogenic and hematotoxic modes of action of benzene are better understood than those for other health effects, such as respiratory, reproduction, or developmental toxicity. However, mechanistic studies show that there may be common initiating factors and key events for different toxic outcomes that may be related to benzene exposure. Current evidence suggests that benzene toxicity is primarily mediated by its metabolites; however, it is not clear which metabolite is the key toxic moiety or whether multiple metabolites contribute to toxicity.
Key events for the mode of action for leukemia were proposed by Meek and Klaunig (2010) and are supported by several lines of experimental and epidemiological evidence. In the initial step, benzene is metabolized, and metabolites are distributed to the bone marrow. Once in the bone marrow, metabolites interact with target cells, forming initiated target bone marrow cells. The mutated cells undergo selective clonal proliferation, which leads to the production of neoplasms, or leukemia. The mode of action for hematotoxicity likely follows the same first steps of metabolism and distribution to the bone marrow, where metabolites interact with hematopoietic cells resulting in altered blood cell populations (Wang et al. 2012).
The mode of action of benzene is thought to be dependent upon its conversion to reactive metabolites by CYP2E1 (ATSDR 2007; Meek and Klaunig 2010). Benzene is readily absorbed into blood and metabolized primarily in the liver, with some secondary metabolism in the bone marrow. Multiple reactive metabolites have been implicated in benzene toxicity, including benzene oxide, phenol, catechol, hydroquinone, and 1,4-benzoquinone. The role of individual metabolites and the precise mechanism of damage to target cells is not clear; however, there are many possibilities.
Benzene metabolites are reported to cause CA, covalent binding, oxidative stress, alteration of gene expression, apoptosis, interference with DNA repair, mediation of epigenetic regulation, and disruption of tumor surveillance (Wang et al. 2012). DNA adducts have been observed in bone marrow and leukocytes in animals and in human hematopoietic cells, which are also the site of MN induction and CA, including aneuploidy, translocation and other structural changes (IARC 2018). Hydroquinone and 1,4-benzoquinone have been implicated in inhibition of DNA repair via inhibition of topoisomerase I and poly(ADP-ribose)polymerase 1 (Luo et al. 2018; Son et al. 2016). Catechol and hydroquinone form superoxide radicals by cycling back and forth with 1,2-benzoquinine and 1,4-benzoquinone forms (North 2020; IARC 2018). The production of radical oxygen species has been implicated in benzene-metabolite induced apoptosis and decreased erythroid differentiation (Sun et al. 2018; Sun et al. 2019; Badham et al. 2010). Phenol, catechol, hydroquinone and 1,4-benzoquinone may also regulate gene expression via epigenetic mechanisms, by altering DNA methylation and miRNA expression profiles (Wang et al. 2012; Ravegnini et al. 2015). Epigenetic regulation results in changes to the expression of cancer-related signalling pathway components, inhibition of DNA repair, apoptosis, and erythroid differentiation (Tian et al. 2012; Yu et al. 2017, 2019; Conti et al. 2016; Gao et al. 2010; Hu et al. 2014; Li et al. 2012, 2013; Liu et al. 2012; Luo et al. 2018; Tabish et al. 2012; Tang et al. 2016). Evidence from animal studies indicates that benzene hematotoxicity is mediated through the aryl hydrocarbon receptor (AhR); however, benzene, hydroquinone and p-benzoquinone do not directly activate AhR (IARC 2018). The varied and overlapping effects of benzene metabolites suggest that multiple metabolites may work together to affect toxic outcomes. The overlap in key molecular events and site of toxicity (bone marrow) leading to hematopoiesis (that is, erythroid differentiation) and leukemias, suggests that hematotoxicity, as well as genotoxicity, may be key precursor events in benzene-induced cancers. However, there is a lack of direct evidence in animals and humans, and causation cannot be concluded at this time.
The mode of action for carcinogenesis is generally considered to be based on a linear concentration-response relationship, although the toxicokinetics and the shape of the exposure-response curve at low (environmental) concentrations is still not clear, as discussed in Section 4.2. Nonetheless, there are an increasing number of proponents of a non-linear, threshold-based mode of action. The Health Council of the Netherlands (2014) concluded that the most likely mode of action is based on genotoxicity and altered gene expression, which are best explained by mechanisms for which a threshold exists. Similarly, North et al. (2020) argued that benzene acts via a non-linear threshold mechanism, primarily because many of the observed mechanisms of genotoxicity are due to indirect DNA damage, which may not disrupt homeostasis at low levels of occurrence. For example, inhibition of topoisomerase can lead to DNA strand breaks, but may also be tolerated at a low level before such breaks occur, suggesting a threshold for the adverse effect. North et al. (2020) also pointed out that Schnatter et al. (2012) found peak exposures may be more relevant to human cancer risk than cumulative exposure (and similar results in animal studies), suggesting that a threshold mechanism may apply to AML and MDS. In a subsequent publication, North et al. (2021) proposed a modified approach, in which risk below a threshold for key events, such as hematotoxicity and genotoxicity (proposed to be 2 ppm [6.4 mg/m3]), is calculated differently than at higher exposures. Below the threshold, a decreasing slope or segmented linear increases are recommended, while above the threshold, a linear increase in AML risk is maintained.
A potential mode of action for reproductive and developmental effects associated with benzene exposure remains unclear. The addition of benzene to in vitro ovarian granulosa cells promoted cell proliferation and apoptosis, reduced cell viability, and altered hormone release (Tarko et al. 2019; Sirotkin et al. 2020). Exposure of human sperm to phenol and hydroquinone caused a significant decline in both sperm motility and viability, as well as loss of sperm nuclear DNA integrity (Mandani et al. 2013). Mihaich and Borgert (2018) argued that endocrine dysfunction was not likely to be the mechanism of benzene-induced reproductive and developmental effects, as the pattern of effects observed in animal studies was inconsistent with those expected from disruption of estrogen, androgen, thyroid, or steroidogenic mechanisms. The US EPA (2002) has suggested several alternative modes of action, including reduced ascorbic acid in the fetus; altered DNA and RNA content in the placenta, fetal liver and maternal liver; damage to peripheral adrenergic nerve fibres; disruption of ovarian blood flow; and reduced uterine steroid production. Direct toxicity to the fetus is also a plausible mode of action, as both benzene and phenol are capable of passing through the placenta. Benzene may also reduce maternal circulation and cause maternal bone marrow depression, resulting in adverse nutritional conditions for the fetus (US EPA 2002). More recently, Sirotkin et al. (2017) proposed that benzene may act on the reproductive system through mechanisms similar to those that have been implicated in hematotoxicity and carcinogenesis, which include chromosome aberration, production of free radicals, effects on cellular metabolism and intracellular protein kinases.
Little is known about a potential mode of action for respiratory toxicity due to benzene. Some evidence suggests that mechanisms similar to those of other endpoints may be involved. Apoptotic changes were observed in the parenchymal components of the lung in rats exposed to benzene (Weaver et al. 2007). In vitro exposure of human alveolar lung epithelial (A549) cells to benzene resulted in a concentration-dependent increase in DNA breaks and radical oxygen species production (Mascelloni et al. 2015; Mögel et al. 2011; Murugesan et al. 2013; Pariselli et al. 2009). Enhanced telomerase activity markers of inflammation were observed in human lung fibroblast cells and A459 cells exposed to low benzene concentrations (Giuliano et al. 2009; Tas et al. 2019). In addition, benzene exposure induced a mutational pattern in tumor suppressor gene TP53 in A549 cells that was similar to those reported in cases of benzene-related human AML (Billet et al. 2010).
5.6 Susceptible and more highly exposed populations
Several populations are more susceptible to benzene due to variations in the way it is metabolized. PBPK modelling suggests that womenFootnote 1 metabolize up to 26% more benzene than men in the same exposure scenario. This is supported by observations in an occupational population, where, although blood concentrations were higher in men during the 5-hour exposure period, benzene was retained far longer in women who had higher blood and exhaled benzene levels 4 hours after the exposure period ended (US EPA 2002; ATSDR 2007; OEHHA 2014). In contrast, personal monitoring studies have reported that menFootnote 1 have higher exposure to benzene, which is attributed to behaviours such as commuting time and time spent outdoors (Wang et al. 2009; Symanski et al. 2009). In animal studies, male mice are more sensitive than females to genotoxic and cellular effects in multiple organs following inhalation exposure to benzene. These differences were attributed to a higher benzene metabolism rate in males, which is thought to be associated with testosterone levels (US EPA 2002). PBPK modelling suggests that, in mice, increasing age may result in decreased elimination of hydroquinone conjugates in older mice, and thus higher benzene-related toxicity (OEHHA 2014).
Many studies have shown that polymorphisms in genes related to benzene metabolism can have a positive or negative effect on individual susceptibility to benzene toxicity. Some polymorphisms in genes including NQO1, GSTT1, GSTM1, MPO, and CYP2E1 showed a statistically significant relationship with an increased risk for adverse effects in benzene-exposed workers (Ramírez‐Lopera 2021). For example, the metabolic enzyme NQO1 maintains quinones in reduced form, such as hydroquinone and catechol, promoting their conjugation and excretion. Polymorphisms that drastically reduce NQO1 production are associated with an increased risk of benzene hematotoxicity, which is also enhanced by increased CYP2E1 activity. It is estimated that 22% of Caucasian and 45% of Asian populations in the US are homozygous for this NQO1 allele (ATSDR 2007). Variants of the CYP2E1 gene have been associated with an increased risk of childhood leukemia (Trafalis et al. 2010). Genetic polymorphisms in the metabolic enzymes GSTT1, NQO1, CYP2E1, and EPHX1 result in changes in the relative levels of the metabolites SPMA, phenol, catechol, and hydroquinone formed by the liver, which would ultimately affect benzene toxicity (OEHHA 2014). Polymorphisms in genes responsible for DNA repair, genomic maintenance, cytokines and chemokines have also been linked to benzene-induced decreases in WBC counts (Wang et al. 2012).
Children may be more susceptible to benzene exposure due to both intrinsic physiological and extrinsic exposure-related factors. Children are expected to have greater exposure to benzene relative to body weight compared to adults, due to increased ventilation rate, increased activity and small size. PBPK modelling suggests that neonates metabolize benzene less efficiently than adults (Valcke and Krishnan 2011). Hematopoietic cell populations undergo maturation and differentiation in infants and children, which may make them susceptible to leukemia induction and hematotoxicity (US EPA 2002). Early exposure may occur as benzene crosses the placental barrier and can be found in cord blood. Nursing infants may also be exposed via breast milk (ATSDR 2007). Epidemiological studies have indicated that exposure to benzene sources during pregnancy or early childhood are associated with increased risk of childhood leukemia. Exposure during pregnancy has also been linked to preterm birth, low birth weight and reduced head circumference. In animal studies, gestational exposure to benzene is fetotoxic and has produced hematotoxic effects in offspring that continued into adulthood.
Populations that are potentially more highly exposed to benzene have been identified in several biomonitoring and personal exposure studies. In CHMS studies, smoking was the strongest predictor of blood benzene concentrations, which were approximately 5-fold higher than those of people who do not smoke. CHMS data also suggest that South Asians have higher exposure to benzene than the general population (Lyonnais-Gagnon et al. 2022; Karthikeyan et al. 2022). In a biomonitoring study of gestational exposure to benzene in a population residing near natural gas extraction activities, self-identifying Indigenous women had significantly higher urinary SPMA levels than non-identifying women (Caron-Beaudoin et al. 2018). Personal exposure studies conducted in the US reported much higher exposures to benzene (and other aromatic VOCs) experienced by Black and Hispanic populations compared to the general population (D'Souza et al. 2009; Symanski et al. 2009). Children excrete higher levels of urinary benzene and metabolites if they live with people who smoke, or live in urban areas (Protano et al. 2010, 2012). Higher blood benzene levels have also been reported among populations that live in urban environments (US CDC 2017).
6.0 Derivation of reference concentrations
The determination of the recommended guideline values is carried out in two stages. First, an RfC is derived by applying uncertainty factors to the concentrations at which the most sensitive non-cancer adverse health endpoint was observed. Alternatively, a risk-specific concentration (RSC) may be selected for a long-term value based on an inhalation unit risk for cancer. The RfC/RSC approach is used for the determination of recommended guideline values to reduce potential health impacts such as those observed in key toxicological, controlled human exposure, and indoor epidemiological studies. For the short-term exposure RfC, the exposure period is specified as one hour. For the long-term exposure RfC or RSC, the exposure is considered to occur over months or years, up to a lifetime.
In the second stage, the short- and long-term exposure RfC and/or RSC are compared with measured exposures in residential indoor air and evaluated with respect to their technical feasibility. In some cases, this may result the guideline value being set at a higher concentration than the RfC and/or RSC (see Section 6.4 for more information). Setting the recommended guideline value at a higher concentration than the RfC and/or RSC results in a smaller margin of exposure between the recommended guideline value and the concentration at which effects have been observed in health studies. Nonetheless, a recommended guideline value derived in this manner does provide a measure of health protection, while remaining an achievable target for improving indoor air quality when evaluating risk management measures.
6.1 Short-term reference concentration
The short-term RfC is intended to apply to brief and/or intermittent exposures and to be representative of peak exposures in an indoor residential setting. Short-term guideline values are recommended to be compared to a one-hour air sample. A short-term RfC is preferentially derived based on studies of short-term, acute, or intermittent exposures in humans that report health effects. If human data are not available, toxicological data may be considered.
Short-term exposure to benzene has been reported to cause respiratory, CNS, hematotoxic, and systematic effects in occupational settings and an accidental environmental release scenario. Exposures in these studies are either multiple orders of magnitude above environmental concentrations (>800 mg/m3 or >800 000 μg/m3), or air concentrations are not reported (for example, in the case of accidental release). In both types of reports, co-exposure to other pollutants is likely. These exposures, as described, last several days to several weeks and are not appropriate to the intended timeline of a brief or intermittent peak exposure. A single controlled human exposure describing health effects for an appropriate time frame reported no symptoms in human volunteers following 2 hours exposure to 350 mg/m3 (110 ppm) (Srbova 1950, as cited in OEHHA 2014). Clinical symptoms were based on a single volunteered remark and were not systematically investigated. The available human studies are therefore not considered appropriate for the derivation of a short-term (1-hour averaging time) RfC for residential indoor exposure.
Toxicological studies of brief and/or intermittent exposures are available in animals. CNS effects, respiratory distress and lethality are reported in response to short exposures to very high concentrations of benzene. However, the benzene concentrations in these studies (that is, >19 000 mg/m3, or 19 000 000 μg/m3) are over 19 000 000-fold higher than indoor residential exposures such as those measured in homes in Canada (mean levels ranging from 0.4 to 2.2 μg/m3). Most values from studies of homes in Canada are based on 24-hour averaging times. One-hour peak values, while expected to be considerably higher than the corresponding 24-hour average, are still expected to be many orders of magnitude below the values measured in the toxicological studies mentioned above. Thus, an RfC derived based on such high exposures is unlikely to be relevant to benzene concentrations found in an indoor residential environment. In addition, repeated or multi-day exposures in animals were identified, but such studies are not considered appropriate for derivation of a short-term (1-hour averaging time) RfC for benzene.
Therefore, a short-term RfC for benzene was not developed due to a lack of studies applicable to the derivation of an RfC for a 1-hour averaging time.
6.2 Long-term reference concentration and risk-specific concentration
6.2.1 Non-cancer long-term reference concentration
Consideration of key studies
The key non-cancer health effects identified for benzene inhalation exposure are respiratory toxicity, reproductive toxicity, and hematotoxicity. Respiratory toxicity and reproductive toxicity are the two main categories of health effects reported at exposure concentrations (1–10 μg/m3[0.5 –3 ppb]) typically found in residential indoor air in Canada. Hematotoxicity has previously been identified as the key effect for non-cancer reference exposure values from other organizations. In humans, hematotoxicity has been observed primarily in occupational settings where "low" exposure is generally 3.2 mg/m3 (1 ppm) or higher.
Key studies of environmentally relevant exposure
Respiratory toxicity was reported in studies of benzene exposure at environmental concentrations in both indoor and outdoor settings. In a cross-sectional study of indoor benzene exposure in Canadian homes, in which the GM concentration was 1.06 μg/m3 (0.33 ppb), an increase in log transformed IQR (1.31 μg/m3, 0.41 ppb) of benzene concentration was negatively associated with FEV1/FVC ratio in males, children under 17, and people aged 39–64 years (Cakmak et al. 2014). A weekly increase of 10 μg/m3 (3.1 ppb) benzene in homes and schools with mean benzene concentrations of 1.0–10.7 μg/m3 (0.31–3.34 ppb) was associated with reduced FEV1, FEV1/FVC and other lung function markers in children in Portugal (Martins et al. 2012). Additionally, benzene exposure in homes with a mean exposure of 3.85 μg/m3 (1.2 ppb) was associated with exacerbation of asthma symptoms in children in a study from France (Rive et al. 2013). However, mixed results have been obtained in indoor studies. Additional studies of indoor air and personal exposure to benzene failed to identify associations between benzene exposure and lung function among asthmatic children, or lower respiratory tract infection, cough, and wheeze in infants (Smargiassi et al. 2014; Ferrero et al. 2017; Vanker et al. 2017). This may be dependent on the effect measured, as a meta-analysis of 15 studies found a statistically significant association between asthma and benzene exposure, but the same study did not find statistically significant associations between benzene exposure and rhinitis or bronchitis (Liu et al. 2022).
Respiratory effects were reported for exposure to much higher concentrations of benzene in animal and human studies. In animal studies, increased respiratory rates and apoptotic changes were reported in parenchymal lung tissue in mice and rats exposed to benzene (OEHHA 2014; Weaver et al. 2007). Occupational exposure to benzene has been linked to reports of shortness of breath and respiratory irritation. Autopsies of individuals with acute lethal exposure to benzene revealed hemorrhagic, edematous lungs, among other effects (ATSDR 2007).
Among the indoor and personal exposure studies, benzene concentrations were highly correlated with other indoor air pollutants that can have similar effects on the respiratory system. Most studies did not adjust for co-pollutants, although smoking was considered in all reported studies (via subject selection or model adjustment). Of the indoor air studies, only Cakmak et al. (2014) adjusted for NO2, O 3 and PM2.5at the clinic site (but not in the home), and Smargiassi et al. (2014) adjusted for the effects of mould. Health effects of benzene exposure have not been assessed in controlled human exposure studies to confirm the specificity of effects like respiratory toxicity and provide more information about the dose response close to the range of environmental exposure. Although the biological plausibility of respiratory toxicity as an effect of benzene is supported by animal and occupational studies, the possibility cannot be excluded that respiratory effects seen in environmental exposure settings may be partially due to co-exposures or mixture effects. However, respiratory toxicity is one of the few health effects associated with benzene that is sufficiently sensitive to be observed at concentrations found in residential indoor settings. Although the current evidence is not appropriate for the development of an RfC, this endpoint should be considered an emerging health effect of concern at environmentally relevant concentrations.
Reproductive and developmental toxicity may be another highly sensitive potential effect of benzene exposure and has been reported in studies of personal and outdoor exposure. Maternal personal exposure to benzene during pregnancy was associated with lower birth weight and smaller newborn head circumference in a study with median benzene levels of 1.8 μg/m3 (0.56 ppb) (Slama et al. 2009). Similar effects on birth weight were associated with increases in maternal outdoor residential benzene exposure in a large case-control study, where the mean ambient benzene concentration was 3.5 μg/m3 (1.1 ppb) (Ghosh et al. 2012). Increases in air benzene concentration of 0.64–1 μg/m3 (0.2–0.31 ppb) during pregnancy were associated with preterm birth in studies with benzene exposure ranging from 1.3–2.1 μg/m3 (0.41–0.66 ppb) (Estarlich et al. 2016; Wilhelm et al. 2011). In addition, a meta-analysis has reported a significant association between benzene exposure and low birthweight, although not between benzene exposure and preterm birth (Liu et al. 2022). Benzene concentrations were highly correlated with those of other traffic and outdoor pollutants in these studies, and similar effects were observed among co-pollutants. Apart from Estarlich et al. (2016), the authors did not consider the effect of co-pollutants, some of which (for example, NO 2 and PM2.5) have been associated with similar reproductive effects (Johnson et al. 2022). Smoking was considered in all studies reviewed (either by subject selection, or model adjustment). Effects reported in toxicology studies support the biological plausibility of reproductive and developmental toxicity as reported in epidemiology studies, although benzene exposure levels were many orders of magnitude higher. As with studies of respiratory effects at environmental exposure concentrations, lack of adjustment for co-exposures and high correlation of similar effects with co-pollutants raise questions about whether the effects are specific to benzene. Although the current evidence is not sufficient to include this endpoint in the derivation of an RfC, reproductive and developmental toxicity is also considered an emerging health effect of concern at environmentally relevant concentrations.
Key studies of occupational exposure
Hematotoxicity is a well-established effect of benzene exposure and may be a precursor to subsequent development of more serious blood conditions. While it is the most sensitive effect at occupational exposure levels, it has rarely been reported at environmental exposure concentrations. No studies were identified that examined the effect of benzene concentrations in air in residential settings (indoor or outdoor) on hematotoxic effects. In animal studies, as in human occupational studies, impaired hematopoietic function demonstrated by changes in circulating blood cells were observed at a wide range of exposures, from <3.2–9 600 mg/m3 (<1–3 000 ppm) (Health Canada 2013a; US EPA 2002; ATSDR 2007). Associations between hematological markers and blood benzene concentration have been reported for populations with heightened exposure due to environmental pollution, as well as for the general population in NHANES and CHMS studies (Chen, Sun et al. 2019; Doherty et al. 2017; Watson et al. 2020; Cakmak et al. 2020). The specificity of hematotoxic effects to benzene exposure has been established in animal studies, as well as in occupational studies where exposure to other hematotoxic agents is frequently addressed in the study design. However, the altered distribution of cell types observed in non-occupationally exposed populations is not consistent with that seen at occupational exposure levels.
As seen in earlier reports, the studies identified in the post-2009 literature search report hematotoxic effects such as declines in cell types derived from myeloid progenitor cells, including granulocytes, platelets and eosinophils in the range of 1 ppm (3.2 mg/m3) benzene (Bassig et al. 2016; Harati et al. 2017). In these, as in older studies, the exposure of control groups is not well characterised—control groups are frequently defined as workers in areas of the workplace or in alternate workplaces with much lower, but not non-existent exposure. Statistically significant effects are invariably observed in the lowest exposure groups, which, in the case of Lan et al. (2004), Bassig et al. (2016) and Harati et al. (2017), are reported as <1 ppm (3.2 mg/m3), without a lower bound. Given the lack of clarity in the identification of a lowest observed adverse effect concentration (LOAEC), the use of a benchmark concentration is an appropriate approach to identify a point of departure. Zhang et al. (2016) conducted benchmark dose modelling of reduced WBC counts in benzene-exposed workers using Hill and Log-probit models, with the lower bounds ranging from 4.4 to 37.8 mg/m3-years (1.4–11.8 ppm-years) of cumulative benzene exposure. This value was selected as the critical effect level and point of departure for hematotoxicity.
Although the biological plausibility and specificity of hematotoxicity to benzene exposure are clear, unresolved questions remain about extrapolating effects at concentrations found in occupational settings to the much lower concentrations typical of indoor residential settings. The specific metabolites associated with hematotoxicity and their relative internal dosimetry at benzene concentrations in residential settings are not clear at this time. This may be reflected in the inconsistency of hematotoxic effects seen in the CHMS and NHANES studies as compared to those reported in occupational settings.
Derivation of reference concentration
Hematotoxic effects were associated with a BMCL ranging from 4.4 to 37.8 mg/m3-years (1.4–11.8 ppm-years) benzene exposure in an occupational study (Zhang et al. 2016). Over a 40-year working life, this is equivalent to a time-weighted average of 0.11–0.95 mg/m3 (0.034–0.3 ppm) benzene. These values are within the range of the points of departure used by ATSDR (2007), ANSES (2008) and OEHHA (2014) to derive long-term inhalation reference exposure levels (See Appendix D, Section D2 for more details).
An adjustment for residential exposure (BMCL* 8 hours/24 hours*5 days/7 days) results in an adjusted BMCL of 26–230 μg/m3 (8.1–72 ppb). An additional adjustment for study length is not required, as the study considered lifelong exposure. An uncertainty factor of 30 was applied to account for the use of healthy workers as subjects (10-fold), and database uncertainty regarding PBPK modelling at environmentally relevant exposures (3-fold). The resulting RfC for hematotoxicity is 0.9 μg/m3 (0.3 ppb).
Thus, 0.9 μg/m3 (0.3 ppb) is adopted as the long-term RfC for non-cancer effects.
6.2.2 Cancer risk-specific concentration
Consideration of key studies and assessments
Benzene is classified as carcinogenic to humans by Health Canada (Environment Canada and Health and Welfare Canada 1993), the US EPA (2002) and IARC (2018), based on strong evidence in humans and experimental animals. Early classification of benzene's carcinogenicity was largely based on occupational studies, and this conclusion is supported by more recent studies involving lower concentrations of benzene. Risk analyses of two key epidemiological cohorts, the Pliofilm and Chinese Worker cohorts, have been conducted by several regulatory agencies and were reviewed in the previous Health Canada Guidance (Health Canada 2013a).
Environment Canada and Health and Welfare Canada (1993) assessed benzene as a priority substance. A study of mortality in the Pliofilm cohort (Rinsky 1987) was considered the most suitable evidence for leukemogenic potency and was the basis for determining a tumorigenic concentration associated with a 5% increase in mortality due to tumors (TC05). Linear extrapolation from the TC 05 gives an air concentration of 3.0 μg/m3 associated with risk level 1 × 10 -5 (Health Canada 2013a).
In the Guidelines for Canadian Drinking Water Quality: Guideline Technical Document – Benzene (2009), Health Canada reviewed the cancer risk assessment conducted by the OEHHA for drinking water (2001). The inhalation cancer risk analysis published by the US EPA in 2000 was reviewed in the previous Health Canada Guidance (Health Canada 2013a). That document concluded that the range of concentrations associated with risks per 1 × 10 -5 from the OEHHA (2001), Health Canada (2009) and US EPA (2000) assessments is 0.6–4.5 μg/m3, and that there is no significant difference between the outcomes of the three assessments. The corresponding range of concentrations for risk per 1 × 10 -6 to 1 × 10 -4 is 0.06–45 μg/m3, with an inhalation unit risk of 1.6 × 10-5–2.2 × 10-6 (μg/m3)-1.
Health Canada published a toxicological reference value (TRV) of 1.6 × 10 -2 (mg/m3) -1 unit risk for inhalation exposure to benzene at federal contaminated sites. The TRV was a unit lifetime leukemia risk to the general population, based on the OEHHA risk analysis (OEHHA 2001) and the analysis in previous Health Canada Guidance (Health Canada 2013a, Health Canada 2021b).
No additional full risk assessments of cancer for benzene were identified that were published since the previous Health Canada assessment of benzene in indoor air (Health Canada 2013a). However, multiple international agencies have published analyses and critiques of previous cancer risk assessments, to support the development of cancer reference values. European authorities have also developed inhalation unit risk values for indoor benzene based on the same cohorts and resulting in a similar range of risk estimates. The range of air concentrations corresponding to a cancer risk level of 10 -5 from these assessments is 1.0–4.5 μg/m3 (see Appendix D, Section D3 for details).
Previous cancer risk assessments used data based on either AML or all leukemias, and linear models of low dose response to benzene exposure. However, some criticism of these approaches is present in the literature. Studies of low-dose exposure in Chinese factory workers led the authors to suggest that benzene metabolism is non-linear at exposures below 9.6 mg/m3 (3 ppm) (Kim et al. 2006a, 2006b). Multiple concerns have been raised about this model. However, if the model were eventually confirmed in future studies, significant changes in the risk estimates from the Pliofilm and other cohorts would be needed (Vlaanderen et al. 2011). Reports of mild benzene-associated hematotoxic effects in general population biomonitoring studies, along with suggestive evidence for benzene-associated carcinogenic effects at environmental exposures (such as clusters of AML in cities with petrochemical industry, and associations between traffic/benzene exposure and childhood leukemia) also indicate a need for a better understanding of benzene metabolism and dose-response at low exposures. In addition to these considerations, recent discussion of the mode of action of benzene carcinogenicity have favoured a threshold-based model of cancer risk, with hematotoxicity and genotoxicity as key precursor events. These discussions are promising, but are just beginning, and at the time of this assessment, a quantitative threshold-based cancer risk assessment was not available from regulatory organizations or in the published literature.
Additionally, Li and Schnatter (2018) have proposed that benzene risk assessment should be based on all MDS, rather than AML or leukemia alone. The authors point out that MDS is likely caused by benzene exposure (although IARC has not drawn this conclusion) as MDS and AML are both neoplastic disorders of the myeloid lineage. MDS is proposed as a more appropriate measure of risk since a) well-conducted epidemiological studies show consistent evidence of excess MDS risk in benzene-exposed workers and b) MDS risk may have been overlooked in previously studied populations, because diagnostic criteria were not as well developed as they are now. However, as MDS is less prevalent than all the leukemias, and may not have been accurately diagnosed in the past, there is significantly less available data on MDS incidence in benzene-exposed populations.
Derivation of a risk-specific concentration
The previous Health Canada Guidance (Health Canada 2013b) concluded that the most reasonable conclusion from key cancer risk assessments was an inhalation unit risk range of 2.2 × 10-6 to 1.6 × 10 -5 (μg/m3)-1, corresponding to concentrations associated with risk at 1 × 10 -5 of 0.6 to 4.5 μg/m3 (Health Canada 2013a). Key issues regarding benzene cancer risk assessment, particularly the shape of the dose-response curve at environmental concentrations, remain unresolved. However, more recent cancer risk assessments also fall into this range, indicating that there is no need to revise the previous conclusion at this time.
The proposed RSC of 0.6 μg/m3 (0.2 ppb) based on cancer risk lies at the conservative end of this scale and corresponds to an inhalation unit risk of 1.6 x 10 -5 (μg/m3)-1.
6.2.3 Selection of a long-term risk-specific concentration
The proposed non-cancer RfC for hematotoxic effects is 0.9 μg/m3 (0.3 ppb). The proposed RSC for cancer risk is 0.6 μg/m3 (0.2 ppb) and is therefore the more health protective of the two values. Some commentary has proposed hematotoxicity as a key initiating event for benzene-related carcinogenicity. However, as many aspects of the mode of action for both hematotoxicity and carcinogenicity are still uncertain, Health Canada does not fully support this conclusion.
Therefore, the proposed long-term RSC for benzene toxicity is 0.6 μg/m3 (0.2 ppb).
6.3 Exposure in Canadian homes in relation to risk-specific concentration and determination of proposed guideline value
In recent years, Health Canada has conducted exposure studies in multiple Canadian cities including Edmonton, Halifax, Regina, Windsor, Ottawa, and Montreal, as well as in the First Nations community of Sioux Lookout Zone, Ontario and a First Nations reserve in southern Manitoba (Health Canada 2010a, 2010b, 2012, 2013b, 2021a; Mallach et al. 2017; Goldberg et al. 2015; Weichenthal et al. 2013; Zhu et al. 2005; Kovesi et al. 2022). These studies are considered the most recent and representative data available for quantifying long-term levels of exposure in Canadian homes. Other Canadian studies using different methods for sampling and analysis support these data (Health Canada 2021a; Li et al. 2019; Héroux et al. 2008; Zhu et al. 2005; National Research Council 2021). Preliminary data suggest that benzene levels in recently built homes are similar to those measured in other homes in Canada (Health Canada 2021a). Long-term RfCs or RSCs are based on the characterization of the concentration-response relationship and, where appropriate, the application of uncertainty factors to account for variability and data gaps. The context within which RfCs or RSCs are to be applied, technical feasibility, and availability of risk mitigation measures are not considered in their determination. However, these issues are relevant to the determination of long-term guideline values.
In order to determine the proposed guideline values, the long-term RfC or RSC is first compared to available exposure data from Canadian homes. The feasibility of achieving the RfC or RSC through the control of indoor sources is then evaluated. If the RfC or RSC is considered feasible, the identified value is set as the proposed guideline value. If not, a higher concentration may be selected, while still targeting a guideline value that is protective of health in consideration of current evidence.
In the present assessment, the criteria guiding the determination of the value for both the proposed short- and long-term guideline values for benzene are:
- a value that is potentially achievable in Canadian homes in the absence of significant sources of indoor benzene; and
- a value that is not associated with appreciable health effects, considering the derived reference exposure levels and currently available evidence.
6.3.1 Long-term risk-specific concentration and proposed guideline value
The long-term RSC for benzene was determined to be 0.6 μg/m3 (0.2 ppb). The range of median indoor air benzene concentrations measured with a 24-hour averaging period in Canadian homes in Health Canada residential indoor air exposure studies was 0.4–2.2 μg/m3, with the 95th percentile ranging from 2.4 to 18.6 μg/m3 (see Table 2). The median indoor benzene concentration in on-reserve homes in Sioux Lookout Zone and southern Manitoba (averaged over 5 or 7 days) ranged from 1.2 to 1.6 μg/m3, with 95th percentiles of 6.3–11.1 μg/m3. Similar benzene concentrations were observed in other Canadian studies that used different sampling and analysis methods (see Section 2.2). These data indicate that the long-term RSC may be exceeded in many Canadian homes. Nonetheless, the RSC was derived using the most recent scientific information and is relevant to indoor air exposure to benzene.
The national average outdoor air concentration for benzene was 0.5 μg/m3 in 2019, which includes rural, urban and high emissions sites. Data from Health Canada studies show that infiltration from outdoor sources is a key source of indoor benzene, and that lower benzene concentrations are associated with a higher AER and open windows. This indicates that ventilation is a key strategy to reduce exposure to benzene in indoor air, with the recognition that the ability of ventilation to reduce indoor benzene concentrations is limited by outdoor concentrations. As the national average outdoor concentration of benzene is lower than the RSC, and lower than the concentration in many homes, ventilation can be considered a viable strategy to achieve a lower benzene concentration indoors. Important sources of benzene in the home include attached garages, smoking indoors, and storage of products such as gasoline, paints and solvents in the garage or home. Data from Health Canada studies also demonstrate that safe storage of gasoline, paints, and solvents, and reduced indoor smoking are associated with lower indoor benzene concentration. Taken together, this data suggests that ventilation and source control can be used to reduce benzene levels below the RSC in many homes in Canada.
Therefore, the proposed long-term guideline value for benzene of 0.6 μg/m3 (0.2 ppb) is considered health protective while also reflecting achievability, given the expected effectiveness of ventilation as a strategy to reduce indoor benzene levels in the absence of a nearby outdoor source of benzene.
7.0 Proposed guidelines
7.1 Proposed guideline value
Guideline value | Concentration | Critical effect(s) | |
---|---|---|---|
μg/m3 | ppb | ||
Long-term | 0.6 | 0.2 | Leukemia |
The proposed guideline value is intended for professional audiences to support evaluation of potential risk and provide justification for risk management actions. When comparing a measured concentration with the long-term guideline value, the sampling time should be at least 24 hours, taken under normal conditions. Moreover, averaging of results of repeated samples taken at different times of the year will provide a more representative estimate of the long-term exposure.
7.2 Risk management recommendations
Many homes in Canada are expected to have indoor benzene concentrations that exceed the long-term guideline value. Exposure to benzene indoors should be limited by ensuring adequate ventilation, controlling, or eliminating indoor sources, and encouraging changes in occupant behaviour. Health Canada does not recommend that individuals measure concentrations of benzene in their home, but instead focus on implementing the risk mitigation strategies discussed below to improve indoor air quality.
In Health Canada studies, several key factors were associated with higher or lower mean indoor benzene concentrations. Indoor benzene concentrations were increased by 40%–240% in homes with an attached garage. Storage of gasoline, paints and solvents in the garage and home was associated with 33%–390% higher concentrations of indoor benzene (Health Canada 2021a; Mallach et al. 2017). In homes where occupants smoked indoors, indoor benzene concentrations were 56%–194% higher depending on the season. In addition to indoor sources, each 10% increase in outdoor benzene concentration was associated with increases of 4.1%–6.4% indoor benzene (Health Canada 2021a). Indoor benzene concentrations were 4%–47% lower in homes with increased ventilation, depending on the ventilation method used, and were 62% lower with the use of an exhaust fan in an attached garage (Health Canada 2021a; Mallach et al. 2017).
In consideration of these observations, several strategies can be employed to reduce indoor exposure to benzene. Many of these measures will also contribute to lowering the concentration of other indoor air contaminants, leading to a general improvement in indoor air quality.
- If you have an attached garage:
- Consider installing and using a garage exhaust fan;
- Ensure that the interface between the attached garage and the home is properly sealed; and
- Avoid idling your car, snow blower, lawnmower, or any gas-powered equipment in the garage.
- Avoid storing gasoline, paint, solvents or other chemicals in the home or attached garage. These products should be stored in tightly sealed containers, and, if possible, kept in a separate building that isn't connected to your home's ventilation system.
- Switch from gasoline-powered home equipment like lawn mowers, leaf blowers and snow blowers to battery-powered equipment.
- Do not smoke indoors.
- Increase ventilation in the home:
- By using natural ventilation strategies like opening windows and interior doors if possible (check the outdoor air quality conditions in your region before opening windows: Air Quality Health Index);
- By using mechanical ventilation strategies like using fans with outside venting, forced air ventilation, energy recovery ventilators (ERVs) and heat recovery ventilators (HRVs); and
- Pay attention to signs that the ventilation rate is too high, such as dry air and drafts.
- For more information, refer to the factsheet: Ventilation and the Indoor Environment (Health Canada 2018) and the infographic: Ventilation and Indoor Air Quality (Health Canada 2019b).
- When outdoor air quality is poor (for example, due to high
levels of traffic during rush hour or wildfire smoke events):
- Keep windows and doors closed, and use air conditioning to maintain a comfortable temperature indoors;
- Properly seal windows and doors with weather stripping; and
- If possible, set your ventilation to recirculate when outdoor air quality is poor and bring in fresh air when outdoor air has improved.
- For more information, refer to the infographic: Protecting your indoor air (Health Canada 2020).
- Ensure that fireplaces and wood stoves are properly installed
and maintained and vent pollutants to the outdoors.
- For more information, refer to Wood smoke and indoor air.
8.0 References
Abplanalp, W., DeJarnett, N., Riggs, D.W., Conklin, D.J., McCracken, J.P., Srivastava, S., Xie, Z., Rai, S., Bhatnagar, A. and O'Toole, T.E. (2017) Benzene exposure is associated with cardiovascular disease risk. PLoS ONE, 12(9): e0183602.
Abplanalp, W.T., Wickramasinghe, N.S., Sithu, S.D., Conklin, D.J., Xie, Z., Bhatnagar, A., Srivastava, S. and O'Toole, T.E. (2019) Benzene Exposure Induces Insulin Resistance in Mice. Toxicological Sciences, 167(2): 426-437.
Amin, M.M., Rafiei, N., Poursafa, P., Ebrahimpour, K., Mozafarian, N., Shoshtari-Yeganeh, B., Hashemi, M. and Kelishadi, R. (2018) Association of benzene exposure with insulin resistance, SOD, and MDA as markers of oxidative stress in children and adolescents. Environmental Science and Pollution Research International, 25(34): 34046-34052.
Amini, H., Yunesian, M., Hosseini, V., Schindler, C., Henderson, S.B. and Künzli, N. (2017) A systematic review of land use regression models for volatile organic compounds. Atmospheric Environment, 171: 1-16.
Angelini, S., Bermejo, J.L., Ravegnini, G., Sammarini, G. and Hrelia, P. (2016) Application of the lymphocyte Cytokinesis-Block Micronucleus Assay to populations exposed to petroleum and its derivatives: Results from a systematic review and meta-analysis. Mutation Research - Reviews in Mutation Research, 770: 58-72.
ANSES (2008) Valeurs guides de qualité d'air intérieur: Le benzène. Available from: https://www.anses.fr/en/system/files/AIR2004etVG004Ra.pdf
Arnold, S.M., Angerer, J., Boogaard, P.J., Hughes, M.F., O'Lone, R.B., Robison, S.H. and Robert Schnatter, A. (2013) The use of biomonitoring data in exposure and human health risk assessment: benzene case study. Critical Reviews in Toxicology, 43(2): 119-153.
ATSDR (2007) Toxicological Profile for Benzene. Agency for Toxic Substances and Disease Registry, Public Health Service, US Department of Health and Human Services, Atlanta, GA. Available from: https://www.atsdr.cdc.gov/ToxProfiles/tp3.pdf
Aurell, J., Gullett, B.K., Tabor, D., Touati, A. and Oudejans, L. (2012) Semivolatile and volatile organic compound emissions from wood-fired hydronic heaters. Environmental Science & Technology, 46(14): 7898-7904.
AGS (Ausschuss für Gefahrstoffe; Comité des substances dangereuses) (2012) Begründung zu Benzol in BekGS910. Stand: Mai 2012. Ausgabe: November 2012. https://www.baua.de/DE/Angebote/Rechtstexte-und-Technische-Regeln/Regelwerk/TRGS/pdf/910/910-benzol.pdf?__blob=publicationFile&v=2. Zugegriffen: 17. Juni 2019
Ayi-Fanou, L., Avogbe, P.H., Fayomi, B., Keith, G., Hountondji, C., Creppy, E.E., Autrup, H., Rihn, B.H. and Sanni, A. (2011) DNA-adducts in subjects exposed to urban air pollution by benzene and polycyclic aromatic hydrocarbons (PAHs) in Cotonou, Benin. Environmental Toxicology, 26(1): 93.
Badham, H.J. and Winn, L.M. (2010) In utero and in vitro effects of benzene and its metabolites on erythroid differentiation and the role of reactive oxygen species. Toxicology and Applied Pharmacology, 244(3): 273-279.
Bari, M.A. and Kindzierski, W.B. (2017) Concentrations, sources and human health risk of inhalation exposure to air toxics in Edmonton, Canada. Chemosphere, 173: 160-171.
Bassig, B.A., Zhang, L., Cawthon, R.M., Smith, M.T., Yin, S., Li, G., Hu, W., Shen, M., Rappaport, S., Barone-Adesi, F., Rothman, N., Vermeulen, R. and Lan, Q. (2014) Alterations in leukocyte telomere length in workers occupationally exposed to benzene. Environmental and Molecular Mutagenesis, 55(8): 673-8.
Bassig, B.A., Zhang, L., Vermeulen, R., Tang, X., Li, G., Hu, W., Guo, W., Purdue, M.P., Yin, S., Rappaport, S.M., Shen, M., Ji, Z., Qiu, C., Ge, Y., Hosgood, H.D., Reiss, B., Wu, B., Xie, Y., Li, L., Yue, F., Freeman, L.E.B., Blair, A., Hayes, R.B., Huang, H., Smith, M.T., Rothman, N. and Lan, Q.(2016) Comparison of hematological alterations and markers of B-cell activation in workers exposed to benzene, formaldehyde and trichloroethylene. Carcinogenesis, 37(7): 692-700.
Batterman, S., Jia, C. and Hatzivasilis, G. (2007) Migration of volatile organic compounds from attached garages to residences: a major exposure source. Environmental Research, 104(2): 224-240.
Batterman, S., Su, F.C., Li, S., Mukherjee, B., Jia, C. and HEI Health Review Committee. (2014) Personal exposure to mixtures of volatile organic compounds: modeling and further analysis of the RIOPA data. Research Report (Health Effects Institute), 181(181): 3-63.
Berr, C., Vercambre, M.N., Bonenfant, S., Singh, Manoux, A., Zins, M. and Goldberg, M. (2010) Occupational exposure to solvents and cognitive performance in the GAZEL cohort: Preliminary results. Dementia and Geriatric Cognitive Disorders, 30(1): 12-19.
Bevan, R., Jones, K., Cocker, J., Assem, F. and Levy, L. (2013) Reference ranges for key biomarkers of chemical exposure within the UK population. International Journal of Hygiene and Environmental Health, 216(2): 170-174.
Billet, S., Paget, V., Garçon, G., Heutte, N., André, V., Shirali, P. and Sichel, F. (2010) Benzene-induced mutational pattern in the tumour suppressor gene TP53 analysed by use of a functional assay, the functional analysis of separated alleles in yeast, in human lung cells. Archives of Toxicology, 84(2): 99-107.
Bird, M.G., Wetmore, B.A., Letinski, D.J., Nicolich, M., Chen, M., Schnatter, A.R. and Whitman, F.T. (2010) Influence of toluene co-exposure on the metabolism and genotoxicity of benzene in mice using continuous and intermittent exposures. Chemico-biological Interactions, 184(1-2): 233-9.
Bogadi-Šare, A., Zavalić, M., Trošić, I., Turk, R., Kontošić, I. and Jelčić, I. (2000) Study of some immunological parameters in workers occupationally exposed to benzene. International Archives of Occupational and Environmental Health, 73(6): 397-400.
Boogaard, P.J. (2017) The low-dose benzene debate needs a sharp blade. Chemico-biological Interactions, 278, 239-241.
Cakmak, S., Dales, R.E., Liu, L., Kauri, L.M., Lemieux, C.L., Hebbern, C. and Zhu, J. (2014) Residential exposure to volatile organic compounds and lung function: Results from a population-based cross-sectional survey. Environmental Pollution, 194: 145-151.
Cakmak, S., Kauri, L.M., Andrade, J. and Dales, R. (2021) Factors influencing volatile organic compounds in Canadian homes. Indoor and Built Environment, 30(8): 1076-1091.
Cakmak, S., Cole, C., Hebbern, C., Andrade, J. and Dales, R. (2020) Associations between blood volatile organic compounds, and changes in hematologic and biochemical profiles, in a population-based study. Environment International, 145: 106121.
Cancelada, L., Sleiman, M., Tang, X., Russell, M.L., Montesinos, V.N., Litter, M.I., Gundel, L.A. and Destaillats, H. (2019) Heated tobacco products: volatile emissions and their predicted impact on indoor air quality. Environmental Science & Technology, 53(13): 7866-7876.
Caron-Beaudoin, E., Ayotte, P., Aker, A., Blanchette, C., Ricard, S., Gilbert, V., Avard, E. and Lemire, M. (2022) Exposure to benzene, toluene and polycyclic aromatic hydrocarbons in Nunavimmiut aged 16 years and over (Nunavik, Canada) - Qanuilirpitaa 2017 survey. Environmental Research, 206: 112586.
Caron-Beaudoin, É., Valter, N., Chevrier, J., Ayotte, P., Frohlich, K. and Verner, M. (2018) Gestational exposure to volatile organic compounds (VOCs) in Northeastern British Columbia, Canada: a pilot study. Environment International, 110: 131-138.
Caron-Beaudoin, E., Whyte, K.P., Bouchard, M.F., Chevrier, J., Haddad, S., Copes, R., Frohlich, K.L., Dokkie, D., Treaty, 8.T., Juul, S., Bouchard, M. and Verner, M.(2022) Volatile organic compounds (VOCs) in indoor air and tap water samples in residences of pregnant women living in an area of unconventional natural gas operations: Findings from the EXPERIVA study. Science of the Total Environment, 805: 150242.
Carugno, M., Pesatori, A.C., Dioni, L., Hoxha, M., Bollati, V., Albetti, B., Byun, H.-N., Bonzini, M., Fustinoni, S., Cocco, P., Satta, G., Zucca, M., Merlo, D.F., Cipolla, M., Bertazzi, P.A. and Baccarelli, A. (2012) Increased mitochondrial DNA copy number in occupations associated with low-dose benzene exposure. Environmental Health Perspectives, 120(2): 210-15.
Charpin, D., Penard-Morand, C., Raherison, C., Kopferschmitt, C., Lavaud, F., Caillaud, D. and Annesi-Maesano, I. (2009) Long-term exposure to urban air pollution measured through a dispersion model and the risk of asthma and allergy in children. Bulletin de l'Academie Nationale de Medecine, 193(6): 1317-28.
Chen, J., Zheng, Z., Chen, Y., Li, J., Qian, S., Shi, Y., Sun, L., Han, Y., Zhang, S. and Yu, K. (2016) Histone deacetylase inhibitors trichostatin A and MCP30 relieve benzene-induced hematotoxicity via restoring topoisomerase IIalpha. PLoS ONE, 11(4): e0153330.
Chen, Q., Sun, H., Zhang, J., Xu, Y. and Ding, Z. (2019) The hematologic effects of BTEX exposure among elderly residents in Nanjing: a cross-sectional study. Environmental Science and Pollution Research, 26(11): 10552-10561.
Chen, L., Guo, P., Zhang, H., Li, W., Gao, C., Huang, Z., Fan, J., Zhang, Y., Li, X., Liu, X., Wang, F., Wang, S., Li, Q., He, Z., Li, H., Chen, S., Wu, X., Ye, L., Li, Q., Tang, H., Wang, Q., Dong, G., Xiao, Y., Chen, W. and Li, D.(2019) Benzene-induced mouse hematotoxicity is regulated by a protein phosphatase 2A complex that stimulates transcription of cytochrome P4502E1. Journal of Biological Chemistry, 294(7): 2486-2499.
Chin, J., Godwin, C., Parker, E., Robins, T., Lewis, T., Harbin, P. and Batterman, S. (2014) Levels and sources of volatile organic compounds in homes of children with asthma. Indoor Air, 24(4): 403-415.
Choi, Y., Kim, J.H., Lee, B. and Hong, Y. (2014) Urinary benzene metabolite and insulin resistance in elderly adults. Science of the Total Environment, 482: 260-268.
Collins, J.J., Anteau, S.E., Swaen, G.M.H., Bodner, K.M. and Bodnar, C.M. (2015) Lymphatic and Hematopoietic Cancers Among Benzene-Exposed Workers. Journal of Occupational and Environmental Medicine, 57(2): 159-63.
Conti, A., Rota, F., Ragni, E., Favero, C., Motta, V., Lazzari, L., Bollati, V., Fustinoni, S. and Dieci, G. (2016) Hydroquinone induces DNA hypomethylation-independent overexpression of retroelements in human leukemia and hematopoietic stem cells. Biochemical and Biophysical Research Communications, 474(4): 691-695.
Cox Jr, L.A., Ketelslegers, H.B. and Lewis, R.J. (2021) The shape of low-concentration dose–response functions for benzene: implications for human health risk assessment. Critical Reviews in Toxicology, 51(2): 95-116.
Cox, L.A., Schnatter, A.R., Boogaard, P.J., Banton, M. and Ketelslegers, H.B. (2017) Non-parametric estimation of low-concentration benzene metabolism. Chemico-biological Interactions, 278: 242-255.
D'Andrea, M.A. and Reddy, G.K. (2014) Health effects of benzene exposure among children following a flaring incident at the British Petroleum Refinery in Texas City. Pediatric Hematology and Oncology, 31(1): 1-10.
DeHate, R.B., Johnson, G.T., and Harbison, R.D. (2011) Risk characterization of vapor intrusion in former manufactured gas plant sites. Regulatory Toxicology and Pharmacology, 9(2): 353-359.
Dimitrova, N.D., Kostadinova, R.Y., Marinova, S.N., Popov, T.A. and Panev, T.I. (2005) Specific immune responses in workers exposed to benzene. International Immunopharmacology, 5(10): 1554-1559.
Doherty, B.T., Kwok, R.K., Curry, M.D., Ekenga, C., Chambers, D., Sandler, D.P. and Engel, L.S. (2017) Associations between blood BTEXS concentrations and hematologic parameters among adult residents of the U.S. Gulf States. Environmental Research, 156: 579-87.
D'Souza J.C., Jia C., Mukherjee B. and Batterman, S. (2009) Ethnicity, housing and personal factors as determinants of VOC exposures. Atmospheric Environment, 43: 2884-2892.
Du, L., Batterman, S., Godwin, C., Rowe, Z. and Chin, J. (2015) Air exchange rates and migration of VOCs in basements and residences. Indoor Air, 25(6): 598-609.
Environment Canada and Health and Welfare Canada (1993) Canadian Environmental Protection Act – Priority Substances List Assessment Report: Benzene. Available from: https://www.canada.ca/content/dam/hc-sc/migration/hc-sc/ewh-semt/alt_formats/hecs-sesc/pdf/pubs/contaminants/psl1-lsp1/benzene/benzene-eng.pdf
Environment and Climate Change Canada (2021) (NAPS database online).
Estarlich, M., Ballester, F., Aguilera, I., Fernandez-Somoano, A., Lertxundi, A., Llop, S., Freire, C., Tardon, A., Basterrechea, M., Sunyer, J. and Iniguez, C. (2011) Residential exposure to outdoor air pollution during pregnancy and anthropometric measures at birth in a multicenter cohort in Spain. Environmental Health Perspectives, 119(9): 1333-8.
Estarlich, M., Ballester, F., Davdand, P., Llop, S., Esplugues, A., Fernandez-Somoano, A., Lertxundi, A., Guxens, M., Basterrechea, M., Tardon, A., Sunyer, J. and Iniguez, C. (2016) Exposure to ambient air pollution during pregnancy and preterm birth: A Spanish multicenter birth cohort study. Environmental Research, 147: 50-8.
Faure, S., Noisel, N., Werry, K., Karthikeyan, S., Aylward, L.L. and St-Amand, A. (2020) Evaluation of human biomonitoring data in a health risk based context: An updated analysis of population level data from the Canadian Health Measures Survey. International Journal of Hygiene and Environmental Health, 223(1): 267-280.
Ferrero, A., Esplugues, A., Estarlich, M., Llop, S., Cases, A., Mantilla, E., Ballester, F. and Iniguez, C. (2017) Infants' indoor and outdoor residential exposure to benzene and respiratory health in a Spanish cohort. Environmental Pollution, 222: 486-94.
Filippini, T., Heck, J.E., Malagoli, C., Giovane, C.D. and Vinceti, M. (2015) A review and meta-analysis of outdoor air pollution and risk of childhood leukemia. Journal of Environmental Science and Health, Part C, 33(1): 36-66.
Filippini, T., Hatch, E.E., Rothman, K.J., Heck, J.E., Park, A.S., Crippa, A., Orsini, N. and Vinceti, M. (2019) Association between outdoor air pollution and childhood leukemia: a systematic review and dose–response meta-analysis. Environmental Health Perspectives, 127(4): 46002.
French, John E., Gatti, Daniel M., Morgan, Daniel L., Kissling, Grace E., Shockley, Keith R., Knudsen, Gabriel A., Shepard, Kim G., Price, Herman C., Deborah, K., Witt, Kristine L., Pedersen, Lars C., Munger, Steven C., Svenson, Karen L. and Churchill, Gary A. (2015) Diversity Outbred Mice Identify Population-Based Exposure Thresholds and Genetic Factors that Influence Benzene-Induced Genotoxicity. Environmental Health Perspectives, 123(3): 237-245.
Gao, A., Zuo, X., Liu, Q., Lu, X., Guo, W. and Tian, L. (2010) Methylation of PARP-1 promoter involved in the regulation of benzene-induced decrease of PARP-1 mRNA expression. Toxicology Letters, 195(2-3): 114-118.
Ghazawi, F.M., Le, M., Cyr, J., Netchiporouk, E., Rahme, E., Alakel, A., Zubarev, A., Powell, M., Moreau, L. and Roshdy, O. (2019) Analysis of acute myeloid leukemia incidence and geographic distribution in Canada from 1992 to 2010 reveals disease clusters in Sarnia and other industrial US border cities in Ontario. Cancer, 125(11): 1886-1897.
Ghosh J.K.C., Wilhelm, M., Su, J., Goldberg, D., Cockburn, M., Jerrett, M. and Ritz, B. (2012) Assessing the influence of traffic-related air pollution on risk of term low birth weight on the basis of land-use-based regression models and measures of air toxics. American Journal of Epidemiology, 175(12): 1262-74.
Giuliano, M., Stellavato, A., Cammarota, M., Lamberti, M., Miraglia, N., Sannolo, N. and De Rosa, M. (2009) Effects of low concentrations of benzene on human lung cells in vitro. Toxicology Letters, 188(2): 130-136.
Glass, D.C., Gray, C.N., Jolley, D.J., Gibbons, C., Sim, M.R., Fritschi, L., Adams, G.G., Bisby, J.A. and Manuell, R. (2003) Leukemia risk associated with low-level benzene exposure. Epidemiology, 14(5): 569-577.
Glass, D.C., Schnatter, A.R., Tang, G., Irons, R.D. and Rushton, L. (2014) Risk of myeloproliferative disease and chronic myeloid leukaemia following exposure to low-level benzene in a nested case–control study of petroleum workers. Occupational and Environmental Medicine, 71(4): 266-274.
Glass, D.C., Gray, C.N., Jolley, D.J., Gibbons, C. and Sim, M.R. (2005) Health Watch exposure estimates: do they underestimate benzene exposure? Chemico-biological Interactions, 153: 23-32.
Goldberg, M.S., Wheeler, A.J., Burnett, R.T., Mayo, N.E., Valois, M., Brophy, J.M. and Giannetti, N. (2015) Physiological and perceived health effects from daily changes in air pollution and weather among persons with heart failure: A panel study. Journal of Exposure Science & Environmental Epidemiology, 25(2): 187-199.
Gong, Z., Li, J., Wang, X., Yu, Y., Ren, M. and Zhou, J. (2019) A meta-analysis of traffic-related air pollution and risk of childhood leukemia. Journal of Pediatric Hematology/Oncology, 41(4): 267-274.
Gordian, M.E., Stewart, A.W. and Morris, S.S. (2010) Evaporative gasoline emissions and asthma symptoms. International Journal of Environmental Research and Public Health, 7(8): 3051-3062.
Harati, B., Shahtaheri, S.J., Karimi, A., Azam, K., Harati, A., Ahmadi, A. and Rad, M.A. (2017) Hematologic evaluation of painting hall workers in an automobile manufacturing company. Shiraz E-Medical Journal, 18(12): e57350.
Hayes, R.B., Yin, S.N., Dosemeci, M., Li, G.L., Wacholder, S., Chow, W.H., Rothman, N., Wang, Y.Z., Dai, T.R., Chao, X.J., Jiang, Z.L., Ye, P.Z., Zhao, H.B., Kou, Q.R., Zhang, W.Y., Meng, J.F., Zho, J.S., Lin, X.F., Ding, C.Y., Li, C.Y., Zhang, Z.N., Li, D.G., Travis, L.B., Blot, W.J. and Linet, M.S. (1996) Mortality among benzene-exposed workers in China. Environmental Health Perspectives, 104 Suppl 6:1349-1352.
Hays, S.M., Pyatt, D.W., Kirman, C.R. and Aylward, L.L. (2012) Biomonitoring Equivalents for benzene. Regulatory Toxicology and Pharmacology, 62(1): 62-73.
Health Canada. (1987) Exposure guidelines for residential indoor air
quality. A report of the
Federal-Provincial Advisory Committee on Environmental and Occupational
Health. Ottawa:
Minister of Supply and Services Canada. Available from: https://publications.gc.ca/site/eng/411621/publication.html
Health Canada (2009) Guidelines for Canadian Drinking Water Quality: Guideline Technical Document – Benzene. Available from: https://www.canada.ca/content/dam/canada/health-canada/migration/healthy-canadians/publications/healthy-living-vie-saine/water-benzene-eau/alt/water-benzene-eau-eng.pdf
Health Canada (2010a) Regina Indoor Air Quality Study 2007: VOC Sampling Data Summary. Health Canada, Ottawa, Ontario, Cat. No.: H128-1/10-617E-PDF.
Health Canada. (2010b) Windsor Ontario Exposure Assessment Study: VOC Sampling Data Summary (2005, 2006). Health Canada, Ottawa, Ontario, Cat. No.: H128-1/10-618E-PDF.
Health Canada. (2012) Halifax Indoor Air Quality Study 2009: Volatile Organic Compounds (VOC) Data Summary. Health Canada, Ottawa, Ontario, Cat. No.: H129-19/2012E-PDF.
Health Canada (2013a). Guidance for Benzene in Residential Indoor Air: Science Assessment Document. Cat No.: H144-14/1-2-2013E-PDF. ISBN: 978-1-100-23131-0. Government of Canada, Ottawa, Canada. Available from: https://www.canada.ca/en/health-canada/services/publications/healthy-living/guidance-benzene-residential-indoor-air.html
Health Canada (2013b) Edmonton Exposure Assessment Study: VOC Sampling Data Summary (2010). Health Canada, Ottawa, Ontario, Cat. No.: H144-15/2013E-PDF.
Health Canada (2015) Third Report on Human Biomonitoring of Environmental Chemicals in Canada: Results of the Canadian Health Measures Survey Cycle 3 (2012-2013) Available from: https://www.canada.ca/content/dam/hc-sc/migration/hc-sc/ewh-semt/alt_formats/pdf/pubs/contaminants/chms-ecms-cycle3/chms-ecms-cycle3-eng.pdf
Health Canada (2017) Fourth Report on Human Biomonitoring of Environmental Chemicals in Canada: Results of the Canadian Health Measures Survey Cycle 4 (2014-2015) Available from: https://www.canada.ca/en/health-canada/services/environmental-workplace-health/reports-publications/environmental-contaminants/fourth-report-human-biomonitoring-environmental-chemicals-canada.html
Health Canada (2018) Factsheet: Ventilation and the Indoor Environment. Government of Canada, Ottawa. Cat.: H144-54/1-2018E-PDF. Available from: https://www.canada.ca/en/health-canada/services/publications/healthy-living/factsheet-ventilation-indoor-environment.html
Health Canada (2019a) Fifth Report on Human Biomonitoring of Environmental Chemicals in Canada: Results of the Canadian Health Measures Survey Cycle 5 (2016-2017) Available from: https://www.canada.ca/en/health-canada/services/environmental-workplace-health/reports-publications/environmental-contaminants/fifth-report-human-biomonitoring.html
Health Canada (2019b) Infographic: Ventilation and Indoor Air Quality. Cat. No.: H144-65/2019-PDF, ISBN: 978-0-0660-31853-0. Available from: https://publications.gc.ca/collections/collection_2019/sc-hc/H144-65-2019-eng.pdf
Health Canada (2020) Infographic: Protecting your Indoor Air from Outdoor Pollutants. Cat. No.: H144-75/2-2020E-PDF, ISBN: 978-0-660-36342-4. Available from: https://www.canada.ca/content/dam/hc-sc/documents/services/publications/healthy-living/protecting-indoor-air-outdoor-pollutants/infographic/infographic-protecting-indoor-air-outdoor-pollutants-eng.pdf
Health Canada (2021a) – personal communication
Health Canada (2021b) Federal Contaminated Site Risk Assessment in Canada: Toxicological Reference Values (TRVs) Version 3.0. Available from: https://publications.gc.ca/collections/collection_2021/sc-hc/H129-108-2021-eng.pdf
Health Canada (2023) Benzene Releases from Gasoline Stations: Implications for Human Health. Available from: https://www.canada.ca/en/health-canada/programs/consultation-benzene-releases-gasoline-stations-implications-human-health/document.html
Health Canada and National Research Council Canada (2019). Volatile organic compounds in new homes: Pilot study report on building product emissions and indoor air quality. Cat.: H144-64/2019E-PDF ISBN: 978-0-660-31604-8, Government of Canada, Ottawa, Canada. Available from: http://publications.gc.ca/pub?id=9.875560&sl=0
Health Council of the Netherlands. Benzene - Health-based recommended occupational exposure limit. The Hague: Health Council of the Netherlands, 2014; publication no. 2014/03.
Herberth, G., Bauer, M., Gasch, M., Hinz, D., Röder, S., Olek, S., Kohajda, T., Rolle-Kampczyk, U., Von Bergen, M. and Sack, U. (2014) Maternal and cord blood miR-223 expression associates with prenatal tobacco smoke exposure and low regulatory T-cell numbers. Journal of Allergy and Clinical Immunology, 133(2): 543-550.
Héroux, M., Gauvin, D., Gilbert, N.L., Guay, M., Dupuis, G., Legris, M. and Lévesque, B. (2008) Housing characteristics and indoor concentrations of selected volatile organic compounds (VOCs) in Quebec City, Canada. Indoor and Built Environment, 17(2): 128-137.
Hoang, T., Castorina, R., Gaspar, F., Maddalena, R., Jenkins, P., Zhang, Q., McKone, T., Benfenati, E., Shi, A. and Bradman, A. (2017) VOC exposures in California early childhood education environments. Indoor Air, 27(3): 609-621.
Hoffman, E., Guernsey, J.R., Walker, T.R., Kim, J.S., Sherren, K. and Andreou, P. (2017) Pilot study investigating ambient air toxics emissions near a Canadian kraft pulp and paper facility in Pictou County, Nova Scotia. Environmental Science and Pollution Research, 24(25): 20685-20698.
Hollins, D.M., Kerger, B.D., Unice, K.M., Knutsen, J.S., Madl, A.K., Sahmel, J.E. and Paustenbach, D.J. (2013) Airborne benzene exposures from cleaning metal surfaces with small volumes of petroleum solvents. International Journal of Hygiene and Environmental Health, 216(3): 324-332.
Hoxha, M., Dioni, L., Bonzini, M., Pesatori, A.C., Fustinoni, S., Cavallo, D., Carugno, M., Albetti, B., Marinelli, B., Schwartz, J., Bertazzi, P.A. and Baccarelli, A. (2009) Association between leukocyte telomere shortening and exposure to traffic pollution: a cross-sectional study on traffic officers and indoor office workers. Environmental Health: a Global Access Science Source, 8: 41.
Hu, J., Ma, H., Zhang, W., Yu, Z., Sheng, G. and Fu, J. (2014) Effects of benzene and its metabolites on global DNA methylation in human normal hepatic L02 cells. Environmental Toxicology, 29(1): 108-116.
Hystad, P., Setton, E., Cervantes, A., Poplawski, K., Deschenes, S., Brauer, M., van Donkelaar, A., Lamsal, L., Martin, R., Jerrett, M. and Demers, P. (2011) Creating national air pollution models for population exposure assessment in Canada. Environmental Health Perspectives, 119(8): 1123-1129.
IARC (1987) Overall evaluations of carcinogenicity: an updating of IARC Monographs Volumes 1 to 42. IARC Monographs on the Evaluation of Carcinogenic Risks to Humans, Suppl, 7: 1–440. PMID: 3482203
IARC (2018) IARC monographs on the evaluation of carcinogenic risks to humans. Volume 120: Benzene. Lyon, France: International Agency for Research on Cancer. Available from: https://publications.iarc.fr/Book-And-Report-Series/Iarc-Monographs-On-The-Identification-Of-Carcinogenic-Hazards-To-Humans/Benzene-2018
Jain, R.B. (2015) Levels of selected urinary metabolites of volatile organic compounds among children aged 6-11 years. Environmental Research, 142: 461-470.
Janitz, A.E., Campbell, J.E., Magzamen, S., Pate, A., Stoner, J.A. and Peck, J.D. (2017) Benzene and childhood acute leukemia in Oklahoma. Environmental Research, 158: 167-173.
Ji, Z., Weldon, R.H., Marchetti, F., Chen, H., Li, G., Xing, C., Kurtovich, E., Young, S., Schmid, T.E., Waidyanatha, S., Rappaport, S., Zhang, L. and Eskenazi, B. (2012) Comparison of aneuploidies of chromosomes 21, X, and Y in the blood lymphocytes and sperm of workers exposed to benzene. Environmental and Molecular Mutagenesis, 53(3): 218-26.
Johnson, M., Shin, H.H., Roberts, E., Sun, L., Fisher, M., Hystad, P., Van Donkelaar, A., Martin, R.V., Fraser, W.D., Lavigne, E., Clark, N., Beaulac, V. and Arbuckle, T.E. (2022) Critical Time Windows for Air Pollution Exposure and Birth Weight in a Multicity Canadian Pregnancy Cohort.Epidemiology (Cambridge, Mass.), 33(1): 7-16.
Kalenge, S., Lebouf, R.F., Hopke, P.K., Rossner, A. and Benedict-Dunn, A. (2013) Assessment of exposure to outdoor BTEX concentrations on the Saint Regis Mohawk Tribe reservation at Akwesasne New York State. Air Quality, Atmosphere & Health, 6(1): 181-193.
Karthikeyan, S., Khoury, C., Pollock, T., Walker, M. and St-Amand, A. Opportunities for the analysis of chemical exposures in racialized populations in Canada: An investigation based on the Canadian Health Measures Survey. (Poster for Health Canada Science Forum 2022).
Kassem, N.O., Kassem, N.O., Jackson, S.R., Liles, S., Daffa, R.M., Zarth, A.T., Younis, M.A., Carmella, S.G., Hofstetter, C.R. and Chatfield, D.A. (2014) Benzene uptake in Hookah smokers and non-smokers attending Hookah social events: regulatory implications. Cancer Epidemiology, Biomarkers & Prevention, 23(12): 2793-2809.
Keller, K.A. and Snyder, C.A. (1988) Mice exposed in utero to 20 ppm benzene exhibit altered numbers of recognizable hematopoietic cells up to seven weeks after exposure. Fundamental and Applied Toxicology, 10(2): 224-232.
Kicinski, M., Saenen, N.D., Viaene, M.K., Den, Hond, E., Schoeters, G., Plusquin, M., Nelen, V., Bruckers, L., Sioen, I., Loots, I., Baeyens, W., Roels, H.A. and Nawrot, T.S. (2016) Urinary t,t-muconic acid as a proxy-biomarker of car exhaust and neurobehavioral performance in 15-year olds. Environmental Research, 151: 521-7.
Kim, S.Y., Son, B., Park, H., Oh, S.H., Lee, J.H., Suh, M. and Park, M.K. (2017) Impact of environmental volatile organic compounds on otitis media in children: Correlation between exposure and urinary metabolites. International Journal of Pediatric Otorhinolaryngology, 93: 157-162.
Kim, S., Vermeulen, R., Waidyanatha, S., Johnson, B.A., Lan, Q., Rothman, N., Smith, M.T., Zhang, L., Li, G. and Shen, M. (2006a) Using urinary biomarkers to elucidate dose-related patterns of human benzene metabolism. Carcinogenesis, 27(4): 772-781.
Kim, S., Vermeulen, R., Waidyanatha, S., Johnson, B.A., Lan, Q., Smith, M.T., Zhang, L., Li, G., Shen, M. and Yin, S. (2006b) Modeling human metabolism of benzene following occupational and environmental exposures. Cancer Epidemiology Biomarkers & Prevention, 15(11): 2246-2252.
Knutsen, J.S., Kerger, B.D., Finley, B. and Paustenbach, D.J. (2013) A calibrated human PBPK model for benzene inhalation with urinary bladder and bone marrow compartments. Risk Analysis, 33(7): 1237-1251.
Koh, D., Chung, E., Jang, J., Lee, H., Ryu, H., Yoo, K., Kim, E. and Kim, K. (2014) Cancer incidence and mortality among temporary maintenance workers in a refinery/petrochemical complex in Korea. International Journal of Occupational and Environmental Health, 20(2): 141-145.
Koh, D., Kim, T., Yoon, Y., Shin, K. and Yoo, S. (2011) Lymphohematopoietic cancer mortality and morbidity of workers in a refinery/petrochemical complex in Korea. Safety & Health at Work, 2(1): 26-33.
Kovesi, T., Mallach, G., Schreiber, Y., McKay, M., Lawlor, G., Barrowman, N., Tsampalieros, A., Kulka, R., Root, A., Kelly, L., Kirlew, M. and Miller, J.D. (2022) Housing conditions and respiratory morbidity in Indigenous children in remote communities in Northwestern Ontario, Canada. CMAJ: Canadian Medical Association Journal = Journal de l'Association Medicale Canadienne, 194(3): E80-E88.
Lan, Q., Zhang, L., Li, G., Vermeulen, R., Weinberg, R.S., Dosemeci, M., Rappaport, S.M., Shen, M., Alter, B.P. and Wu, Y. (2004) Hematotoxicity in workers exposed to low levels of benzene. Science, 306(5702): 1774-1776.
Lee, J.H., Lee, H.S., Park, M.R., Lee, S.W., Kim, E.H., Cho, J.B., Kim, J., Han, Y., Jung, K. and Cheong, H.K. (2014) Relationship between indoor air pollutant levels and residential environment in children with atopic dermatitis. Allergy, Asthma & Immunology Research, 6(6): 517-524.
Levy, I., Mihele, C., Lu, G., Narayan, J. and Brook, J.R. (2014) Evaluating multipollutant exposure and urban air quality: pollutant interrelationships, neighborhood variability, and nitrogen dioxide as a proxy pollutant. Environmental Health Perspectives, 122(1): 65-72.
Li, W., and Schnatter, A.R. (2018) Benzene risk assessment: does new evidence on myelodysplastic syndrome justify a new approach? Critical Reviews in Toxicology, 48(6): 417-432.
Li, X., Wu, X., Xue, M., Wang, Y., Wang, J., Li, Y., Zhang, G. and Yi, Z. (2012) The role of DNA methylation in catechol-enhanced erythroid differentiation of K562 cells. Toxicology and Applied Pharmacology, 265(1): 43-50.
Li, Y., Wu, X., Li, X., Yu, C., Li, Y. and Yi, Z. (2013) Changes in DNA methylation of erythroid-specific genes in K562 cells exposed to phenol and hydroquinone. Toxicology, 312: 108-114.
Li, Y., Cakmak, S. and Zhu, J. (2019) Profiles and monthly variations of selected volatile organic compounds in indoor air in Canadian homes: Results of Canadian national indoor air survey 2012–2013. Environment International, 126: 134-144.
Li, J., Zhang, X., He, Z., Sun, Q., Qin, F., Huang, Z., Zhang, X., Sun, X., Liu, L., Chen, L., Gao, C., Wang, S., Wang, F., Li, D., Zeng, X., Deng, Q., Wang, Q., Zhang, B., Tang, H., Chen, W. and Xiao, Y. (2017) MGMT hypomethylation is associated with DNA damage in workers exposed to low-dose benzene. Biomarkers, 22(5): 470-5.
Lim, S.K., Shin, H.S., Yoon, K.S., Kwack, S.J., Um, Y.M., Hyeon, J.H., Kwak, H.M., Kim, J.Y., Kim, T.H. and Kim, Y.J. (2014) Risk assessment of volatile organic compounds benzene, toluene, ethylbenzene, and xylene (BTEX) in consumer products. Journal of Toxicology and Environmental Health, Part A, 77(22-24): 1502-1521.
Linet, M.S., Yin, S.-N., Gilbert, E.S., Dores, G.M., Hayes, R.B., Vermeulen, R., Tian, H.-Y., Lan, Q., Portengen, L., Ji, B.-T., Li, G.-L. and Rothman, N. (2015) A retrospective cohort study of cause-specific mortality and incidence of hematopoietic malignancies in Chinese benzene-exposed workers. International Journal of Cancer, 137(9): 2184-97.
Liu, N., Bu, Z., Liu, W., et al. (2022) Health effects of exposure to indoor volatile organic compounds from 1980 to 2017: A systematic review and meta-analysis. Indoor Air. 32: e13038
Liu, C., Huang, X. and Li, J. (2020) Outdoor benzene highly impacts indoor concentrations globally. Science of the Total Environment, 720: 137640.
Liu, L., Ling, X., Liang, H., Gao, Y., Yang, H., Shao, J. and Tang, H. (2012) Hypomethylation mediated by decreased DNMTs involves in the activation of proto-oncogene MPL in TK6 cells treated with hydroquinone. Toxicology Letters, 209(3): 239-245.
Logue, J.M., Sleiman, M., Montesinos, V.N., Russell, M.L., Litter, M.I., Benowitz, N.L., Gundel, L.A. and Destaillats, H. (2017) Emissions from electronic cigarettes: assessing vapers' intake of toxic compounds, secondhand exposures, and the associated health impacts. Environmental Science & Technology, 51(16): 9271-9279.
Luo, H., Liang, H., Chen, Y., Chen, S., Xu, Y., Xu, L., Liu, J., Zhou, K., Peng, J. and Guo, G. (2018) miR-7-5p overexpression suppresses cell proliferation and promotes apoptosis through inhibiting the ability of DNA damage repair of PARP-1 and BRCA1 in TK6 cells exposed to hydroquinone. Chemico-biological Interactions, 283: 84-90.
Lyonnais-Gagnon, P., Malowany, M., Noisel, N. and St-Amand, A. Mesures des concentrations de benzène dans l'air intérieur et analyse des facteurs d'exposition influençant la concentration du benzène sanguin dans la population canadienne. (Manuscript in preparation).
MacNeill, M., Dobbin, N., St‐Jean, M., Wallace, L., Marro, L., Shin, T., You, H., Kulka, R., Allen, R.W. and Wheeler, A.J. (2016) Can changing the timing of outdoor air intake reduce indoor concentrations of traffic‐related pollutants in schools? Indoor Air, 26(5): 687-701.
Majumdar, D., Dutta, C. and Sen, S. (2016) Inhalation exposure or body burden? Better way of estimating risk – An application of PBPK model. Environmental Toxicology and Pharmacology, 41: 54-61.
Mallach, G., St‐Jean, M., MacNeill, M., Aubin, D., Wallace, L., Shin, T., Van Ryswyk, K., Kulka, R., You, H. and Fugler, D. (2017) Exhaust ventilation in attached garages improves residential indoor air quality. Indoor Air, 27(2): 487-499.
Mandani, P., Desai, K. and Highland, H. (2013) Cytotoxic effects of benzene metabolites on human sperm function: an in vitro study. ISRN Toxicology, 2013: 397524.
Manoukian, A., Buiron, D., Temime-Roussel, B., Wortham, H. and Quivet, E. (2016) Measurements of VOC/SVOC emission factors from burning incenses in an environmental test chamber: influence of temperature, relative humidity, and air exchange rate. Environmental Science and Pollution Research, 23(7): 6300-6311.
Martin, K.A., Lin, E.Z., Hilbert, T.J., Godri Pollitt, K.J. and Haynes, E.N. (2021) Survey of airborne organic compounds in residential communities near a natural gas compressor station: Response to community concern. Environmental Advances, 5: 100076.
Martins, P.C., Valente, J., Papoila, A.L., Caires, I., Araujo-Martins, J., Matae, P., Lopes, M., Torres, S., Rosado-Pinto, J., Borrego, C., Annesi-Maesano, I. and Neuparth, N. (2012) Airways changes related to air pollution exposure in wheezing children. European Respiratory Journal, 39(2): 246-53.
Mascelloni, M., Delgado-Saborit, J.M., Hodges, N.J. and Harrison, R.M. (2015) Study of gaseous benzene effects upon A549 lung epithelial cells using a novel exposure system. Toxicology Letters, 237(1): 38-45.
McNally, K., Sams, C., Loizou, G. and Jones, K. (2017) Evidence for non-linear metabolism at low benzene exposures? A reanalysis of data. Chemico-biological Interactions, 278: 256-268.
Medinsky, M., Sabourin, P., Lucier, G., Birnbaum, L. and Henderson, R. (1989) A toxikinetic model for simulation of benzene metabolism. Experimental Pathology, 37(1-4): 150-154.
Meek, M.B. and Klaunig, J.E. (2010) Proposed mode of action of benzene-induced leukemia: Interpreting available data and identifying critical data gaps for risk assessment. Chemico-biological Interactions, 184(1-2): 279-285.
Mihaich, E.M. and Borgert, C.J. (2018) Hypothesis-driven weight-of-evidence analysis for the endocrine disruption potential of benzene. Regulatory Toxicology and Pharmacology, 100: 7-15.
Miller, L., Xu, X., Grgicak-Mannion, A., Brook, J. and Wheeler, A. (2012) Multi-season, multi-year concentrations and correlations amongst the BTEX group of VOCs in an urbanized industrial city. Atmospheric Environment, 61: 305-315.
Miller, L., Xu, X., Wheeler, A., Atari, D.O., Grgicak-Mannion, A. and Luginaah, I. (2011) Spatial variability and application of ratios between BTEX in two Canadian cities. The Scientific World Journal, 11: 2536-2549.
Mögel, I., Baumann, S., Böhme, A., Kohajda, T., von Bergen, M., Simon, J. and Lehmann, I. (2011) The aromatic volatile organic compounds toluene, benzene and styrene induce COX-2 and prostaglandins in human lung epithelial cells via oxidative stress and p38 MAPK activation. Toxicology, 289(1): 28-37.
Morales, E., Garcia-Esteban, R., de la Cruz, O.A., Basterrechea, M., Lertzundi, A., de Dicastillo, M.D., Zabaleta C. and Sunyer, J. (2015) Intrauterine and early postnatal exposure to outdoor air pollution and lung function at preschool age. Thorax, 70(1): 64-73.
Murugesan, K., Baumann, S., Wissenbach, D.K., Kliemt, S., Kalkhof, S., Otto, W., Mögel, I., Kohajda, T., von Bergen, M. and Tomm, J.M. (2013) Subtoxic and toxic concentrations of benzene and toluene induce Nrf2‐mediated antioxidative stress response and affect the central carbon metabolism in lung epithelial cells A549. Proteomics, 13(21): 3211-3221.
Mustafa, N., Mumford, K.G., Gerhard, J.I., and O'Carroll, D.M. (2014) A three-dimensional numerical model for linking community-wide vapour risks. Journal of Contaminant Hydrology, 156: 38-51.
National Research Council Canada (2021) – personal communication
NPRI National Pollutant Release Inventory (2022) Ottawa (ON): Government of Canada. Search results for benzene. [Accessed May 2022]. https://pollution-waste.canada.ca/national-release-inventory/
North, C.M., Rooseboom, M., Kocabas, N.A., Schnatter, A.R., Faulhammer, F. and Williams, S.D. (2020) Modes of action considerations in threshold expectations for health effects of benzene. Toxicology Letters, 334: 78-86.
North, C.M., Schnatter, A.R., Rooseboom, M., Kocabas, N.A., Dalzell, A. and Williams, S.D. (2021) Key event-informed risk models for benzene-induced acute myeloid leukaemia. Toxicology Letters, 340: 141-152.
OEHHA (2001) Public Health Goal for Benzene in Drinking Water. Available from: https://oehha.ca.gov/water/public-health-goal/public-health-goal-benzene-drinking-water
OEHHA (2014) Benzene Reference Exposure Levels Technical Support Document for the Derivation of Noncancer Reference Exposure Levels Appendix D1. Available from: https://oehha.ca.gov/media/downloads/crnr/benzenerelsjune2014.pdf
Olsson, A., Togawa, K., Schuz, J., Le Cornet, C., Fervers, B., Oksbjerg Dalton, S., Pukkala, E., Feychting, M., Skakkebaek, N.E. and Hansen, J. (2018) Parental occupational exposure to solvents and heavy metals and risk of developing testicular germ cell tumors in sons (NORD-TEST Denmark). Scandinavian Journal of Work, Environment and Health, 44(6): 658-69.
Pariselli, F., Sacco, M., Ponti, J. and Rembges, D. (2009) Effects of toluene and benzene air mixtures on human lung cells (A549). Experimental and Toxicologic Pathology, 61(4): 381-386.
Peluso, M., Munnia, A., Ceppi, M., Giese, R.W., Catelan, D., Rusconi, F., Godschalk, R.W.L. and Biggeri, A. (2013) Malondialdehyde-deoxyguanosine and bulky DNA adducts in schoolchildren resident in the proximity of the Sarroch industrial estate on Sardinia Island, Italy. Mutagenesis, 28(3): 315-21.
Petry, T., Vitale, D., Joachim, F.J., Smith, B., Cruse, L., Mascarenhas, R., Schneider, S. and Singal, M. (2014) Human health risk evaluation of selected VOC, SVOC and particulate emissions from scented candles. Regulatory Toxicology and Pharmacology, 69(1): 55-70.
Pickworth, W.B., Rosenberry, Z.R., Yi, D., Pitts, E.N., Lord-Adem, W. and Koszowski, B (2018) Cigarillo and little cigar mainstream smoke constituents from replicated human smoking. Chemical Research in Toxicology, 31(4): 251-258.
Price, P.S., Rey, T.D., Fontaine, D.D. and Arnold, S.M. (2012) A reanalysis of the evidence for increased efficiency in benzene metabolism at airborne exposure levels below 3 ppm. Carcinogenesis, 33(11): 2094-2099.
Protano, C., Guidotti, M., Manini, P., Petyx, M., La Torre, G. and Vitali, M. (2010) Benzene exposure in childhood: role of living environments and assessment of available tools. Environment International, 36(7): 779-787.
Protano, C., Andreoli, R., Manini, P., Guidotti, M. and Vitali, M. (2012) A tobacco-related carcinogen: assessing the impact of smoking behaviours of cohabitants on benzene exposure in children. Tobacco Control, 21(3): 325-329.
Rahman, M.M. and Kim, K. (2014) Potential hazard of volatile organic compounds contained in household spray products. Atmospheric Environment, 85:266-274.
Ramírez‐Lopera, V., Uribe‐Castro, D., Bautista‐Amorocho, H., Silva‐Sayago, J.A., Mateus‐Sánchez, E., Ardila‐Barbosa, W.Y. and Pérez‐Cala, T.L. (2021) The effects of genetic polymorphisms on benzene‐exposed workers: A systematic review. Health Science Reports, 4(3): e327.
Rappaport, S.M., Kim, S., Lan, Q., Li, G., Vermeulen, R., Waidyanatha, S., Zhang, L., Yin, S., Smith, M.T. and Rothman, N. (2010) Human benzene metabolism following occupational and environmental exposures. Chemico-biological Interactions, 184(1-2): 189-195.
Rappaport, S.M., Kim, S., Thomas, R., Johnson, B.A., Bois, F.Y. and Kupper, L.L. (2013) Low-dose metabolism of benzene in humans: science and obfuscation. Carcinogenesis, 34(1): 2-9.
Rappaport, S.M., Kim, S., Lan, Q., Vermeulen, R., Waidyanatha, S., Zhang, L., Li, G., Yin, S., Hayes, R.B., Rothman, N. and Smith, M.T. (2009) Evidence that humans metabolize benzene via two pathways. Environmental Health Perspectives, 117(6): 946-952.
Ravegnini, G., Sammarini, G., Hrelia, P. and Angelini, S. (2015) Key genetic and epigenetic mechanisms in chemical carcinogenesis. Toxicological Sciences, 148(1): 2-13.
Rinsky, R., Hornung, R., Silver, S. and Tseng, C. (2002) Benzene exposure and hematopoietic mortality: A long‐term epidemiologic risk assessment. American Journal of Industrial Medicine, 42(6): 474-480.
Rinsky, R.A., Smith, A.B., Hornung, R., Filloon, T.G., Young, R.J., Okun, A.H. and Landrigan, P.J. (1987) Benzene and leukemia. New England Journal of Medicine, 316(17): 1044-1050.
Rinsky, R.A., Young, R.J. and Smith, A.B. (1981) Leukemia in benzene workers. American Journal of Industrial Medicine, 2(3): 217-245.
Rive, S., Hulin, M., Baiz, N., Hassani, Y., Kigninlman, H., Toloba, Y., Caillaud, D. and Annesi-Maesano, I. (2013) Urinary S-PMA related to indoor benzene and asthma in children. Inhalation Toxicology, 25(7): 373-82.
RIVM (2001) Rijksinstituut Voor Volksgezondheid En Milieu National Institute of Public Health and the Environment. RIVM Report 711701025. Baars, A.J. et al. 2001. Re-evaluation of human-toxicological maximum permissible risk levels. Bilthoven, Netherlands.
RIVM (2007) Centre for Inspection Research, Emergency Response and
Drinking
Water. RIVM Report 609021044/2007. Dusseldorp, A. et al. 2007.
Health-based guideline values for the indoor environment. Bilthoven,
Netherlands.
Rothman, N., Li, G.L., Dosemeci, M., Bechtold, W.E., Marti, G.E., Wang, Y.Z., Linet, M., Xi, L.Q., Lu, W., Smith, M.T. and Titenko‐Holland, N. (1996) Hematotoxocity among Chinese workers heavily exposed to benzene. American journal of industrial medicine, 29(3): 236-246.
Rozen, M.G., Snyder, C.A. and Albert, R.E. (1984) Depressions in B- and T-lymphocyte mitogen-induced blastogenesis in mice exposed to low concentrations of benzene. Toxicology letters, 20(3): 343-349.
Ruchirawat, M., Navasumrit, P. and Settachan, D. (2010) Exposure to benzene in various susceptible populations: Co-exposures to 1,3-butadiene and PAHs and implications for carcinogenic risk. Chemico-biological Interactions, 184(1-2): 67-76.
Rushton, L., Schnatter, A.R., Tang, G. and Glass, D.C. (2014) Acute myeloid and chronic lymphoid leukaemias and exposure to low-level benzene among petroleum workers. British Journal of Cancer, 110(3): 783-7.
Sabbath, E.L., Glymour, M.M., Berr, C., Singh-Manoux, A., Zins, M., Goldberg, M. and Berkman, L.F. (2012) Occupational solvent exposure and cognition: does the association vary by level of education? Neurology, 78(22): 1754-60.
Sabbath, E.L., Gutierrez, L.A., Okechukwu, C.A., Singh-Manoux, A., Amieva, H., Goldberg, M., Zins, M. and Berr, C. (2014) Time may not fully attenuate solvent-associated cognitive deficits in highly exposed workers. Neurology, 82(19): 1716-1723.
Schlink, U., Thiem, A., Kohajda, T., Richter, M. and Strebel, K. (2010) Quantile regression of indoor air concentrations of volatile organic compounds (VOC). Science of the Total Environment, 408(18): 3840-3851.
Schnatter, A.R., Glass, D.C., Tang, G., Irons, R.D. and Rushton, L. (2012) Myelodysplastic syndrome and benzene exposure among petroleum workers: an international pooled analysis. Journal of the National Cancer Institute, 104(22): 1724-1737.
Schnatter, A.R., Kerzic, P.J., Zhou, Y., Chen, M., Nicolich, M.J., Lavelle, K., Armstrong, T.W., Bird, M.G., Lin, L. and Fu, H. (2010) Peripheral blood effects in benzene-exposed workers. Chemico-Biological Interactions, 184(1-2): 174-181.
Schnatter, A.R., Rooseboom, M., Kocabas, N.A., North, C.M., Dalzell, A., Twisk, J., Faulhammer, F., Rushton, E., Boogaard, P.J. and Ostapenkaite, V. (2020) Derivation of an occupational exposure limit for benzene using epidemiological study quality assessment tools. Toxicology Letters, 334: 117-144.
Scholten, B., Vlaanderen, J., Stierum, R., Portengen, L., Rothman, N., Lan, Q., Pronk, A. and Vermeulen, R. (2020) A quantitative meta-analysis of the relation between occupational benzene exposure and biomarkers of cytogenetic damage. Environmental Health Perspectives, 128(8): 087004.
Schripp, T., Salthammer, T., Wientzek, S. and Wensing, M. (2014) Chamber studies on nonvented decorative fireplaces using liquid or gelled ethanol fuel. Environmental Science & Technology, 48(6): 3583-3590.
Sekar, A., Varghese, G.K. and Varma, M.R. (2019) Analysis of benzene air quality standards, monitoring methods and concentrations in indoor and outdoor environment. Heliyon, 5(11): e02918.
Setton, E.M., Veerman, B., Erickson, A., Deschenes, S., Cheasley, R., Poplawski, K., Demers, P.A. and Keller, C.P. (2015) Identifying potential exposure reduction priorities using regional rankings based on emissions of known and suspected carcinogens to outdoor air in Canada. Environmental Health, 14(1): 1-16.
Sheu, R., Stönner, C., Ditto, J.C., Klüpfel, T., Williams, J., Gentner, D.R. (2020) Human transport of thirdhand tobacco smoke: A prominent source of hazardous air pollutants into indoor nonsmoking environments. Science Advances 6(10): eaay4109.
Sirotkin, A.V., Fabian, D., Babel'ová, J., Vlčková, R., Alwasel, S. and Harrath, A.H. (2017) Metabolic state can define the ovarian response to environmental contaminants and medicinal plants. Applied Physiology, Nutrition, and Metabolism, 42(12): 1264-1269.
Sirotkin, A., Záhoranska, Z., Tarko, A., Popovska-Percinic, F., Alwasel, S. and Harrath, A.H. (2020) Plant isoflavones can prevent adverse effects of benzene on porcine ovarian activity: an in vitro study. Environmental Science and Pollution Research, 27(23): 29589-29598.
Slama, R., Thiebaugeorges, O., Goua, V., Aussel, L., Sacco, P., Bohet, A., Forhan, A., Ducot, B., Annesi-Maesano, I., Heinrich, J., Magnin, G., Schweitzer, M., Kaminski, M., Charles, M.A. and EDEN Mother-Child Cohort Study Group. (2009) Maternal personal exposure to airborne benzene and intrauterine growth. Environmental Health Perspectives, 117(8): 1313-1321.
Smargiassi, A., Goldberg, M.S., Wheeler, A.J., Plante, C., Valois, M., Mallach, G., Kauri, L.M., Shutt, R., Bartlett, S. and Raphoz, M. (2014) Associations between personal exposure to air pollutants and lung function tests and cardiovascular indices among children with asthma living near an industrial complex and petroleum refineries. Environmental Research, 132: 38-45.
Smith, M.T. (2010) Advances in understanding benzene health effects and susceptibility. Annual Review of Public Health, 31: 133-48.
Son, M.Y., Deng, C.X., Hoeijmarkers, J.H., Rebel, V.I. and Hasty, P. (2016) A mechanism for 1,4-Benzoquinone-induced genotoxicity. Oncotarget, 7(29): 46433-46447.
Statistics Canada (2022) Table 16-10-0041-01 Chemicals and synthetic resins production, annual. DOI: https://doi.org/10.25318/1610004101-eng [Accessed May 2022]
Staudt, A.M., Whitworth, K.W., Chien, L.-C., Whitehead, L.W. and Gimeno Ruiz de Porras, D. (2019) Association of organic solvents and occupational noise on hearing loss and tinnitus among adults in the U.S., 1999-2004. International Archives of Occupational and Environmental Health, 92(3): 403-13.
Stenehjem, J.S., Kjærheim, K., Bråtveit, M., Samuelsen, S.O., Barone-Adesi, F., Rothman, N., Lan, Q. and Grimsrud, T.K. (2015) Benzene exposure and risk of lymphohaematopoietic cancers in 25 000 offshore oil industry workers. British Journal of Cancer, 112(9): 1603-1612.
St-Jean, M., St-Amand, A., Gilbert, N.L., Soto, J.C., Guay, M., Davis, K. and Gyorkos, T.W. (2012) Indoor air quality in Montréal area day-care centres, Canada. Environmental Research, 118: 1-7.
Stroud, C.A., Zaganescu, C., Chen, J., McLinden, C.A., Zhang, J. and Wang, D. (2016) Toxic volatile organic air pollutants across Canada: multi-year concentration trends, regional air quality modelling and source apportionment. Journal of Atmospheric Chemistry, 73(2): 137-164.
Sun, R., Meng, X., Pu, Y., Sun, F., Man, Z., Zhang, J., Yin, L. and Pu, Y. (2019) Overexpression of HIF-1a could partially protect K562 cells from 1, 4-benzoquinone induced toxicity by inhibiting ROS, apoptosis and enhancing glycolysis. Toxicology In Vitro, 55: 18-23.
Sun, S., Zhang, C., Gao, J., Qin, Q., Zhang, Y., Zhu, H., Yang, X., Yang, D. and Yan, H. (2018) Benzoquinone induces ROS-dependent mitochondria-mediated apoptosis in HL-60 cells. Toxicology and Industrial Health, 34(4): 270-281.
Symanski, E., Stock, T.H., Tee, P.G. and Chan, W. (2009) Demographic, residential, and behavioral determinants of elevated exposures to benzene, toluene, ethylbenzene, and xylenes among the US population: results from 1999–2000 NHANES. Journal of Toxicology and Environmental Health, Part A, 72(14): 903-912.
Tabish, A.M., Poels, K., Hoet, P. and Godderis, L. (2012) Epigenetic factors in cancer risk: effect of chemical carcinogens on global DNA methylation pattern in human TK6 cells. PloS ONE, 7(4): e34674.
Talibov, M., Lehtinen-Jacks, S., Martinsen, J.I., Kjaerheim, K., Lynge, E., Sparen, P., Tryggvadottir, L., Weiderpass, E., Kauppinen, T., Kyyronen, P. and Pukkala, E. (2014) Occupational exposure to solvents and acute myeloid leukemia: A population-based, case-control study in four Nordic countries. Scandinavian Journal of Work, Environment and Health, 40(5): 511-7.
Tang, K., Yu, C., Jiang, L., Gong, M., Liu, W., Wang, Y., Cui, N., Song, W., Sun, Y. and Yi, Z. (2016) Long-term exposure of K562 cells to benzene metabolites inhibited erythroid differentiation and elevated methylation in erythroid specific genes. Toxicology Research, 5(5): 1284-1297.
Tarko, A., Štochmal'ová, A., Jedličková, K., Hrabovszká, S., Vachanová, A., Harrath, A.H., Alwasel, S., Alrezaki, A., Kotwica, J. and Baláži, A. (2019) Effects of benzene, quercetin, and their combination on porcine ovarian cell proliferation, apoptosis, and hormone release. Archives Animal Breeding, 62(1): 345-351.
Taş, İ., Zhou, R., Park, S., Yang, Y., Gamage, C.D., Son, Y., Paik, M. and Kim, H. (2019) Inflammatory and tumorigenic effects of environmental pollutants found in particulate matter on lung epithelial cells. Toxicology in Vitro, 59: 300-311.
TCEQ (2015) Benzene – Development Support Document. Available from: https://www.tceq.texas.gov/downloads/toxicology/dsd/final/benzene.pdf
Tian, J., Peng, C., Yu, X., Yang, X. and Yan, H. (2012) Expression and methylation analysis of p15 and p16 in mouse bone marrow cells exposed to 1, 4-benzoquinone. Human & Experimental Toxicology, 31(7): 718-725.
Trafalis, D.T., Panteli, E.S., Grivas, A., Tsigris, C. and Karamanakos, P.N. (2010) CYP2E1 and risk of chemically mediated cancers. Expert Opinion on Drug Metabolism & Toxicology, 6(3): 307-319.
Umweltbundesamt (German Environment Agency) (2020) Vorläufiger Leitwert für Benzol in der Innenraumluft. Bundesgesundheitsblatt 63: 361–367 (2020). Available from: https://doi.org/10.1007/s00103-019-03089-4
US CDC (2017) Centers for Disease Control and Prevention. National Biomonitoring Program: Biomonitoring Summary – Benzene. Updated April 7, 2017. Available from: https://www.cdc.gov/biomonitoring/Benzene_BiomonitoringSummary.html
US EPA (2000) Integrated Risk Information System (IRIS) Chemical Assessment Summary: Benzene: CASRN 71-43-2. United States Environmental Protection Agency, Washington, D.C.
US EPA (2002) Toxicological Review of Benzene (Noncancer Effects) (CAS No. 71-43-2). In Support of Summary Information on the Integrated Risk Information System (IRIS). October 2002, United States Environmental Protection Agency, Washington, D.C.
Uzma, N., Kumar, B.S. and Hazari, M.A.H. (2010) Exposure to benzene induces oxidative stress, alters the immune response and expression of p53 in gasoline filling workers. American Journal of Industrial Medicine, 53(12): 126-70.
Valcke, M. and Krisnan, K. (2011) Assessing the impact of the duration and intensity of inhalation exposure on the magnitude of the variability of internal dose metrics in children and adults. Inhalation Toxicology, 23(14): 863-77.
Vanker, A., Barnett, W., Workman, L., Nduru, P.M., Sly, P.D., Gie, R.P. and Zar, H.J. (2017) Early-life exposure to indoor air pollution or tobacco smoke and lower respiratory tract illness and wheezing in African infants: a longitudinal birth cohort study. The Lancet Planetary Health, 1(8): e328.
Vardoulakis, S., Giagloglou, E., Steinle, S., Davis, A., Sleeuwenhoek, A., Galea, K.S., Dixon, K. and Crawford, J.O. (2020) Indoor exposure to selected air pollutants in the home environment: A systematic review. International Journal of Environmental Research and Public Health, 17(23): 8972.
Vaughan Watson, C., Naik, S., Lewin, M., Ragin-Wilson, A. and Irvin-Barnwell, E. (2021) Associations between select blood VOCs and hematological measures in NHANES 2005–2010.Journal of Exposure Science & Environmental Epidemiology, 31(2): 366-376.
Villeneuve, P.J., Jerrett, M., Brenner, D., Su, J., Chen, H. and McLaughlin, J.R. (2014) A case-control study of long-term exposure to ambient volatile organic compounds and lung cancer in Toronto, Ontario, Canada. American Journal of Epidemiology, 179(4): 443-451.
Vinceti, M., Rothman, K.J., Crespi, C.M., Sterni, A., Cherubini, A., Guerra, L., Maffeis, G., Ferretti, E., Fabbi, S. and Teggi, S. (2012) Leukemia risk in children exposed to benzene and PM10 from vehicular traffic: a case–control study in an Italian population. European Journal of Epidemiology, 27(10): 781-790.
Vlaanderen, J., Portengen, L., Rappaport, S.M., Glass, D.C., Kromhout, H. and Vermeulen, R. (2011) The impact of saturable metabolism on exposure-response relations in 2 studies of benzene-induced leukemia. American Journal of Epidemiology, 174(5): 621-629.
Wang, L., He, X., Bi, Y. and Ma, Q. (2012) Stem cell and benzene-induced malignancy and hematotoxicity. Chemical Research in Toxicology, 25(7): 1303-1315.
Wang, S-W., Majeed, M.A., Chu, P-L, and Lin, H-C. (2009) Characterizing relationships between personal exposures to VOCs and socioeconomic, demographic, behavioral variables. Atmospheric Environment, 43:2296-2302.
Wathier, L., Venet, T., Bonfanti, E., Nunge, H., Cosnier, F., Parietti-Winkler, C., Campo, P. and Pouyatos, B. (2019) Measuring the middle-ear reflex: A quantitative method to assess effects of industrial solvents on central auditory pathways. Neurotoxicology, 74: 58-66.
Weaver, C., Liu, S., Lu, J. and Lin, B. (2007) The effects of benzene exposure on apoptosis in epithelial lung cells: localization by terminal deoxynucleotidyl transferase-mediated dUTP-biotin nick end labeling (TUNEL) and the immunocytochemical localization of apoptosis-related gene products. Cell Biology and Toxicology, 23(3): 201-220.
Weichenthal, S., Mallach, G., Kulka, R., Black, A., Wheeler, A., You, H., St‐Jean, M., Kwiatkowski, R. and Sharp, D. (2013) A randomized double‐blind crossover study of indoor air filtration and acute changes in cardiorespiratory health in a First Nations community. Indoor Air, 23(3): 175-184.
Wentworth, G.R., Aklilu, Y., Landis, M.S. and Hsu, Y. (2018) Impacts of a large boreal wildfire on ground level atmospheric concentrations of PAHs, VOCs and ozone. Atmospheric Environment, 178: 19-30.
Wheeler, A.J., Smith-Doiron, M., Xu, X., Gilbert, N.L. and Brook, J.R. (2008) Intra-urban variability of air pollution in Windsor, Ontario—measurement and modeling for human exposure assessment. Environmental Research, 106(1): 7-16.
Wheeler, A.J., Wong, S.L., Khoury, C. and Zhu, J. (2013) Predictors of indoor BTEX concentrations in Canadian residences. Health Reports, 24(5): 11-17.
WHO (2000) Evaluation of risks to human health – benzene. In: Air Quality Guidelines for Europe. 2nd ed. Copenhagen (DK): WHO Regional Office for Europe.
Wichmann, F.A., Muller, A., Busi, L.E., Cianni, N., Massolo, L., Schlink, U., Porta, A. and Sly, P.D. (2009) Increased asthma and respiratory symptoms in children exposed to petrochemical pollution. Journal of Allergy and Clinical Immunology, 123(3): 632-8.
Wilhelm, M., Ghosh, J.K., Su, J., Cockburn, M., Jerrett, M. and Ritz, B. (2011) Traffic-related air toxics and preterm birth: a population-based case-control study in Los Angeles County, California. Environmental Health, 10(1): 1-12.
Williams, A.D., Grantz, K.L., Zhang, C., Nobles, C., Sherman, S. and Mendola, P. (2019) Ambient Volatile Organic Compounds and Racial/Ethnic Disparities in Gestational Diabetes Mellitus: Are Asian/Pacific Islander Women at Greater Risk? American Journal of Epidemiology, 188(2): 389-97.
Won D., Nong G., Yang W. and Collins P. (2014). Available from: Material emissions testing: VOCs from wood, paint, and insulation materials.National Research Council, Ottawa, ON.
Won D., Nong G., Yang W. and Lusztyk E. (2015). Available from: VOC emissions from evaporative sources in residential garages. National Research Council, Ottawa, ON.
Wong, O., Harris, F., Armstrong, T.W. and Hua, F. (2010) A hospital-based case-control study of acute myeloid leukemia in Shanghai: analysis of environmental and occupational risk factors by subtypes of the WHO classification. Chemico-biological Interactions, 184(1-2): 112-128.
Xiong, Y., Bari, M.A., Xing, Z. and Du, K. (2020) Ambient volatile organic compounds (VOCs) in two coastal cities in western Canada: Spatiotemporal variation, source apportionment, and health risk assessment. Science of the Total Environment, 706: 135970.
Yao, Y., Pennell, K. G. and Suuberg, E. (2011). Vapor intrusion in urban settings: effect of foundation features and source location. Procedia environmental sciences, 4:245–250.
Yeager, R., Riggs, D.W., DeJarnett, N., Srivastava, S., Lorkiewicz, P., Xie, Z., Krivokhizhina, T., Keith, R.J., Srivastava, S. and Browning, M.H. (2020) Association between residential greenness and exposure to volatile organic compounds. Science of the Total Environment, 707; 135435.
Yin, S., Hayes, R.B., Linet, M.S., Li, G., Dosemeci, M., Travis, L.B., Li, C., Zhang, Z., Li, D. and Chow, W. (1996) A cohort study of cancer among benzene‐exposed workers in China: overall results. American Journal of Industrial Medicine, 29(3): 227-235.
Yin, S.N., Li, G.L., Tain, F.D., Fu, Z.I., Jin, C., Chen, Y.J., Luo, S.J., Ye, P.Z., Zhang, J.Z. and Wang, G.C. (1987) Leukaemia in benzene workers: a retrospective cohort study. British Journal of Industrial Medicine, 44(2): 124-128.
Yu, C., Cui, N., Wang, Y., Wang, Y., Liu, W., Gong, M., Zhao, X., Rong, L. and Yi, Z. (2017) Changes in DNA methylation of erythroid-specific genes in K562 cells exposed to catechol in long term. Toxicology in Vitro, 43: 21-28.
Yu, C., Li, Y., Zhao, X., Yang, S., Li, L., Cui, N., Rong, L. and Yi, Z. (2019) Benzene metabolite 1, 2, 4-benzenetriol changes DNA methylation and histone acetylation of erythroid-specific genes in K562 cells. Archives of Toxicology, 93(1): 137-147.
Zhang, L., Lan, Q., Ji, Z., Li, G., Shen, M., Vermeulen, R., Guo, W., Hubbard, A.E., McHale, C.M., Rappaport, S.M., Hayes, R.B., Linet, M.S., Yin, S., Smith, M.T. and Rothman, N. (2012) Leukemia-related chromosomal loss detected in hematopoietic progenitor cells of benzene-exposed workers. Leukemia, 26(12): 2494-8.
Zhang, G., Ji, B., Li, Y., Zheng, G., Ye, L., Hao, Y., Ren, J., Zhou, L., Xu, X., Zhu, Y. and Xia, Z. (2016) Benchmark Doses Based on Abnormality of WBC or Micronucleus Frequency in Benzene-Exposed Chinese Workers. Journal of Occupational & Environmental Medicine, 58(2): e39-44.
Zhou, C., Baiz, N., Banerjee, S., Charpin, D.A., Caillaud, D., De Blay, F., Raherison, C., Lavaud, F. and Annesi-Maesano, I. (2013) The relationships between ambient air pollutants and childhood asthma and eczema are modified by emotion and conduct problems. Annals of Epidemiology, 23(12): 778-83.
Zhu, J., Newhook, R., Marro, L. and Chan, C.C. (2005) Selected volatile organic compounds in residential air in the city of Ottawa, Canada. Environmental Science & Technology, 39(11): 3964-3971.
Zhu, J., Wong, S.L. and Cakmak, S. (2013) Nationally representative levels of selected volatile organic compounds in Canadian residential indoor air: population-based survey. Environmental Science & Technology, 47(23): 13276-13283.
Appendix A: List of acronyms and abbreviations
- AER
- air exchange rate
- ANSES
- Agence Nationale de Sécurité sanitaire de l'Alimentation, de l'Environnement et du Travail (France)
- AML
- acute myeloid leukemia
- ANLL
- acute non-lymphocytic leukemia
- ATSDR
- Agency for Toxic Substances and Disease Registry (US)
- BE
- biomonitoring equivalent
- BMCL
- lower bound of benchmark concentration
- BTEX
- benzene, toluene, ethylbenzene and xylenes
- CA
- chromosomal aberrations
- CHMS
- Canadian Health Measures Survey
- CI
- confidence interval
- CML
- chronic myeloid leukemia
- CNS
- central nervous system
- CYP2E1
- cytochrome P450 2E1
- DNA
- deoxyribonucleic acid
- EPHX1
- epoxide hydrolase 1
- ERV
- energy recovery ventilator
- FEF
- forced expiratory flow
- FEV1
- forced expiratory volume (1s)
- FVC
- forced vital capacity
- GM
- geometric mean
- GST
- glutathione-S-transferase
- HOMA-IR
- homeostatic model assessment for insulin resistance
- HRV
- heat recovery ventilator
- IARC
- International Agency for Research on Cancer
- I/O
- indoor-to-outdoor ratio
- IQR
- interquartile range
- LOAEC
- lowest observed adverse effect concentration
- MCHC
- mean corpuscular hemoglobin concentration
- MDS
- myelodysplastic syndromes
- MN
- micronuclei
- MPD
- myeloproliferative disorder
- MRL
- minimal risk level
- NHANES
- National Health and Nutrition Examination Survey
- NHL
- non-Hodgkin lymphoma
- NO2
- nitrogen dioxide
- NOAEC
- no observed adverse effect concentration
- NQO1
- NAD(P)H: quinone dehydrogenase 1
- OEHHA
- Office of Environmental Health Hazard Assessment (California)
- OR
- odds ratio
- PBPK
- physiologically based pharmacokinetic
- ppb
- parts per billion
- ppm
- parts per million
- RBC
- red blood cell
- REL
- reference exposure level
- RfC
- reference concentration
- RIAQG
- Residential Indoor Air Quality Guidelines
- RIVM
- National Institute for Public Health and the Environment (the Netherlands)
- RNA
- ribonucleic acid
- RR
- relative risk
- RSC
- risk-specific concentration
- SCE
- sister chromatid exchange
- SPMA
- S-phenylmercapturic acid
- TC 05
- tumorigenic concentration (5%)
- TCEQ
- Texas Commission on Environmental Quality
- TRV
- toxicological reference value
- t,t-MA
- trans, trans-muconic acid
- US EPA
- United States Environmental Protection Agency
- VOC
- volatile organic compound
- WBC
- white blood cell
- WHO
- World Health Organization
Appendix B: Human exposure studies
Study | Participants | Exposure | Results | NOAEC/ LOAEC |
---|---|---|---|---|
D'Andrea et al. 2014 | Retrospective study of the adverse health effects of 312 children (157 exposed to benzene) from a flaring incident at the British Petroleum in Texas City. Children included in the study were under the age of 17. | Exposure to benzene was determined by identifying residential areas that were affected by the flaring incident at the refinery that released 7 000 kg of benzene and other contaminants. Children in these residential areas were chosen to be a part of the study. The exposure to benzene from the incident lasted up to 40 days. Children outside of the affected residential areas were included as controls in the study. | In comparison to the controls(7.3 ± 1.7 x 103/µL), exposed children showed significantly decreased WBCs(6.8 ± 2.1×103/µL)(P= 0.02). Exposed children also had significantly increased mean platelet counts (278.4 ± 59.9 x 103/µL) compared to the controls (261.6±51.7 x 103/µL) (P=0.005). Higher liver enzyme values were found in the exposed group compared to the control group. Serum ALP levels were 183.7 ± 95.6 IU/L in exposed participants compared to the unexposed (165 ± 70.3 IU/L) (p = 0.04). Mean AST levels were significantly higher in the exposed group (23.6 ± 15.3 IU/L) compared to the unexposed group (20.5 ±5.5 IU/L) (p = 0.005). Increased levels of mean serum ALT were observed in the exposed group (19.2± 7.8) compared to the unexposed group (16.9±6.9) (p = 0.005). | Not determined. |
Study | Participants | Exposure | Results | NOAEC/ LOAEC |
---|---|---|---|---|
Cakmak et al. 2014 | Cross-sectional study of the Canadian population (CHMS Cycle 2); 3 039 participants between 6 and 79 years of age; both sexes; among people who do not smoke. | Indoor benzene air concentrations were measured in homes intended to be representative of the Canadian population. Benzene and 83 other VOCs were measured in residences as 7-day passive samples. The geometric mean concentration of benzene was 1.06 μg/m3 (log-transformed IQR was 1.31 μg/m3). | Benzene was negatively associated with lung function (percent change in FEV1/FVC (95% CI) associated with an IQR change in benzene = -0.98 (-1.76, -0.20)). FEV1/FVC was significantly impaired in males (Relative Risk (RR) (95% CI) = -1.75 (-2.71, -0.79)) but not females (RR (95% CI = -0.01 (-0.55, 0.57)). Percent change in FEV1/FVC associated with an IQR change in benzene concentration was also observed in individuals below 17 years of age (RR (95% CI) = -2.3 (-3.7, -0.9) and people 39 to 64 years of age ([RR (95% CI) = -1.45 (-2.44, -0.45]), but not in people 17 to 38 years of age ([RR (95% CI) = -0.15 (-1.11, 0.82]). Benzene was among 10 VOCs (others were decanal, 2-furancarboxaldehyde, hexanal, nonanal, octanal, styrene, a-pinene, 2-methyl-1,2-butadiene and naphthalene) that were negatively associated with lung function. | LOAEC for decreased lung function is oneIQR increase in indoor benzene (1.31 μg/m3). |
Martins et al. 2012 | Prospective panel study of 51 schoolchildren (28 boys and 23 girls; average age = 7.3 years) | Indoor air benzene was measured in schools and homes four times using diffusive samplers (one sample during each 2 winter seasons and 2 summer seasons). In the houses, the benzene level was highest in the winter with mean values of 3.3 μg/m3 in the first winter and 11.9 μg/m3 in the second winter, while summer values were 1.1 and 1.8 μg/m3, respectively. Mean school values were appreciably lower, ranging from 1.4 to 5.0 μg/m3 in the winter and 0.5 to 1.2 μg/m3 in the summer. Total exposure in homes and schools was estimated to be 2.9 to 10.7 μg/m3 in the winter and 1.0 to 1.6 μg/m3 in the summer. | The authors concluded that a benzene increase of 10 μg/m3 (3.3 ppb) per week was significantly associated with a 4.33% decrease in FEV1(95% CI, -7.13 to -1.13%), 1.17% decrease in FEV1/FVC (95% CI, -3.24 to -0.18%), 5.89% decrease in FEF25-75%(95% CI, -10.16 to -1.62%), 2.79% increased change in FEV 1 (DFEV1) (95% CI, 0.92–4.65%), and decreased acidity of exhaled breath condensate (an indicator of airway inflammation). Models were adjusted for parental smoking and other sociodemographic, home and physical characteristics, but not for co-pollutants. Increasing total exposures to PM10, NO2, toluene and ethylbenzene were also associated with a decrease in lung function in these children. | LOAEC for clinical respiratory outcomes in children = a weekly 10 μg/m3 increase in the indoor air concentration in schools or residences (these involve somewhat different exposure patterns). |
Rive et al. 2013 | Case-control study of benzene and other VOCs/ PM2.5in homes of 32 asthmatic and 31 non-asthmatic children (mean age about 14 years). | Benzene measured in indoor air of homes. Median benzene concentration was 3.85 μg/m3 in asthmatic homes and 1.9 μg/m3 in non-asthmatic homes. | ORs of 8.11 for asthma (95% CI, 1.41 to 46.43) and 10.10 for being woken by wheezing (95% CI, 2.06 to 49.78) were reported for children with exposure above the sample median. ORs were greater than 1 but not significant for wheezing during the last 12 months (OR = 1.89); dry cough at night (OR = 1.25); wheezing in chest after an effort in the last 12 months (OR = 10.38); and more than 4 wheezing crises in the last year (OR = 2.95). Models were adjusted for exposure to passive smoking, atopy, family allergy, age, and sex, but not for co-pollutants. | LOAEC for increased asthma symptoms = 3.85 μg/m3 in residential indoor air |
Smargiassi et al. 2014 | A 10-day panel study of 72 children (from non-smoking homes) with asthma 7–12 years of age living in proximity to an industrial complex housing two refineries in Montreal, Quebec. | The children carried a small backpack for personal monitoring of benzene (collected using Summa canisters) and other air pollutants. Personal concentrations of benzene in the participants were: -Median (25th–75th percentile): 2.10 (1.26–3.67) μg/m3 |
Regardless of model adjustment, exposure to benzene was not associated with a decline in lung function or cardiovascular effects in this group of children after adjustments for parental smoking and other confounders. Co-pollutants were not adjusted for in this study. | Not determined. |
Ferrero et al. 2017 | Prospective cohort study from Spain of 708 newborns during their first year of life. | Residential indoor and outdoor concentrations of benzene were measured using passive samplers during a 15-day period. Residences were grouped as urban, metropolitan, semi-urban, residential area and rural. Median indoor air concentrations ranged from 1.23 to 2.38 μg/m3 (rural had the highest). Outdoor median air concentrations were lower, ranging from 0.52 to 1.21 μg/m3 (metropolitan had the highest). Air concentrations were also grouped according to season, and winter tended to have the greatest indoor concentrations. | Respiratory endpoints including persistent cough, low respiratory tract infections, and wheezing in infants, evaluated using a questionnaire administered to parents, were not associated with residential benzene exposure levels. Parental smoking was adjusted for in the study but not co-pollutants. | Not determined. |
Vanker et al. 2017 | Longitudinal birth cohort study of 1 065 infants in South Africa. | Indoor air benzene concentrations were measured using diffusion tubes in each home for 2 weeks: once during pregnancy (antenatal sample) and once within 4 to 6 months after infant's birth (postnatal). Median benzene concentration was 4.29 μg/m3 for antenatal samples and 3.12 μg/m3 for postnatal samples. | When the incidence of lower respiratory tract infection (LRTI) or wheezing was compared between infants living in homes with benzene levels above the South Africa indoor air standard of 5 μg/m3 and those exposed to levels below the standard, no significant increase in LRTI was found. Parental smoking was adjusted for in the study but not co-pollutants. | NOAEC for increased LRTI or wheezing either pre- or post-natal: 5 μg/m3 as an indoor residential concentration |
Wichmann et al. 2009 | Cross-sectional study of 181 children (ages 6–12 years) living close to petrochemical plants (n = 52), urban region with heavy traffic (n = 37), semi-rural area (n=63) and a residential area (n = 30) in Argentina. | Outdoor median benzene concentrations were 19.3 μg/m3 in the industrial area; 2.9 μg/m3 in the urban area; 1.9 μg/m3 in the semi-rural area; and 5.4 μg/m3 in the residential area. | Children living close to the industrial area had significantly increased asthma symptoms (all symptoms; p <0.001) and significantly decreased lung function tests (FEV1, FEV1/FVC and FEF25-75; all p <0.001). ORs for a 1 μg/m3 increase in benzene were significantly increased for all lung function tests: FEV 1 = 1.19; FVC = 1.07; FEV1/FVC = 1.26; FEF25-75= 1.22; BDR = 1.07. Models were adjusted for parental smoking but not for ambient co-pollutants. | LOAEC for increased asthma symptoms and decreased lung functions = a benzene increase of 1 μg/m3 as an outdoor residential concentration. |
Morales et al. 2015 | Population-based cohort study of 620 mother-child pairs from Spain. | Outdoor residential benzene concentrations were estimated using regional measured concentrations and area-specific land-use regression models. Mean benzene concentrations in outdoor air was 0.83 μg/m3. | During the second trimester and using the adjusted model results, benzene concentrations were associated with a RR of 1.22 for decreased FEV 1 < 80% (p = 0.027) for an IQR increase in benzene of 0.28 μg/m3. Models were adjusted for parental smoking but not for ambient co-pollutants. Similar effects were found for NO2. | LOAEC for decreased lung function in preschoolers = 0.28 μg/m3 during pregnancy in residential outdoor air. |
Charpin et al. 2009 | Cross-sectional study of 4 907 children aged from 9 to 11 years living in France. | Outdoor benzene concentrations were estimated using dispersion models. The low exposure group had a median benzene level of 1.7 μg/m3 while the high exposure group had a median benzene level of 3.0 μg/m3. | Prevalence rates of asthma, exercise-induced hyper-reactivity, atopic dermatitis and pollen sensitization were correlated with benzene and at least one of the following substances: SO2, PM10, NOx, NO 2 and CO. The highest OR was for asthma for exposure to benzene over the last year (1.41) (p <0.01). Models were adjusted for parental smoking but not for ambient co-pollutants. | Not determined. |
Zhou et al. 2013 | Cross-sectional study of 4209 children (age 10–12 years, 2 097 boys and 2 112 girls) in France. | Outdoor benzene concentrations at school address were estimated using dispersion modelling. Mean benzene concentration was estimated to be 2.41 μg/m3. | For an IQR increase from 1.73 to 2.92 μg/m3 in children with emotional and behavioural problems, significant increases in adjusted ORs were reported for wheezing (OR = 1.22; p <0.01), doctor-diagnosed asthma (OR = 1.18; p = 0.01) and eczema (OR = 1.48; p <0.01). Models were adjusted for parental smoking but not for ambient co-pollutants. All other substances evaluated (that is, CO, NO2, NOx, PM 10 and total VOCs) also showed similarly elevated ORs. | LOAEC for increased wheezing, asthma and eczema = 2.92 μg/m3 in outdoor air at school address. |
Study | Participants | Exposure | Results | NOAEC/ LOAEC |
---|---|---|---|---|
Olsson et al. 2018 | Case control study of 3 421 male participants (sons of fathers/mothers who may have been occupationally exposed to benzene) diagnosed with testicular germ cell tumours (TGCT) in Denmark from 1981 to 2014. Controls (n = 14 024) were from the central population registry and matched for year of birth. | Exposure of fathers/mothers was determined by the job exposure matrix for Denmark (NOCCA-DANJEM). Groups were defined as exposed or unexposed | No benzene-specific analysis. The associations of TGCT with maternal or paternal occupational exposure to solvents or heavy metals were null. | Not determined. |
Slama et al. 2009 | 271 non-smoking pregnant women recruited in two French maternity hospitals as part of a mother–child cohort. | Personal exposure measured using a diffusive air sampler for a week during the 27th gestational week. Median benzene exposure was 1.8 μg/m3 (5th, 95th percentiles, 0.5, 7.5 μg/m3). Categorical benzene exposure (μg/m3): < 1.4; 1.4–2.59; ≥ 2.6 |
Birth weight: Each increase of one in log-transformed benzene exposure was associated with an adjusted decrease of 68 g in birth weight (95% CI, -135 to -1 g). (Results were not statistically significant when analyzed categorically). Head circumference at birth: a decrease of 1.9 mm observed for each increase by one in log-transformed benzene exposure (95% CI, –3.8 to 0.0). When analyzed categorically, results were only borderline significant at the highest exposure level (≥ 2.6 μg/m3): 3.7 mm (95% CI, –7.3 to 0.0 mm). Head circumference during pregnancy: a decrease in head circumferences were observed but they are mostly statistically non-significant. Regression models were adjusted for confounders including those related to physical measurements of the fetus and mother at week 27, maternal occupational exposure to paint and pesticides, and maternal passive smoking. Models were not adjusted for co-pollutants. |
Not determined. |
Ghosh et al. 2012 | Case-control study of 8 191 term, low birth weight and 370 922 normal weight children born in Los Angeles County within a 5-mile radius of the California Air Resources Board Monitoring station. Other co-pollutants were evaluated including other VOCS, NOx, CO, etc. | Benzene concentrations were measured outside from the California monitoring station. Mean benzene concentration was 1.1 ppb (3.5 μg/ m3) and the IQR was 0.8 ppb (2.6 μg/ m3) | Exposure to benzene (mean = 3.5 μg/m3) during the third trimester was found to be associated with a borderline significant OR of 1.03 (95% CI, 1.00 to 1.05) per IQR (2.6 μg/m3, 0.8 ppm) increase for term low birth weight in infants. Models were adjusted for gestational age, maternal age, and socioeconomic factors, but not for smoking or co-pollutants. Similar associations were also reported for toluene, ethylbenzene, and xylenes. | LOAEC for low birth weight = increase of 2.6 μg/m3 |
Estarlich et al. 2011 | Prospective multicentre cohort study of 2 337 pregnant women. NO 2 was another pollutant evaluated. | Benzene concentrations in residential outdoor air were measured based on passive samples for 7 days. The mean benzene concentration was 1.6 μg/m3 for the four regions; the greatest regional concentration was 2.3 μg/m3. | No associations between weight, length, and head circumference for one- and two-pollutant models for a 1 μg/m3 increase in benzene concentrations. A two-pollutant model was adjusted to consider NO 2 separately from benzene. All models were adjusted for smoking, maternal age, pregnancy weight, paternal height, gestational weight gain, and other factors. | NOAEC for anthropometric measure birth outcomes: 1 μg/m3 increase in residential outdoor air concentration. |
Wilhelm et al. 2011 | Case-control study of 241 415 births from June 2004 to March 2006 residing within a 5-mile radius from the Southern California Air Quality Management District Multiple Air Toxics Exposure Study (MATES III) monitoring station. This study sought to examine the risks of preterm births in Los Angeles women exposed to high levels of traffic-related air pollutants. The preterm cases were n = 10 265 and n = 10 265 based on gestational age at birth. | The residential locations of the births were determined from birth certificates, LUR models were used to assign exposure to each pollutant. For benzene exposure, 24-hour averages were collected every 3 days from the MATES monitoring services within five miles of each woman's residence. Data was averaged over different pregnancy periods based on the birth date and gestational age reported on the birth certificates. IQR for benzene for the entire pregnancy was 0.20 ppb. | An increased risk of preterm birth (OR = 1.09, 95% CI, 1.06–1.13) per IQR (0.64 μg/m3, 0.20 ppb) increase in traffic-related outdoor benzene exposure during the entire pregnancy was reported. Benzene exposure was positively correlated with the following pollutants: NO, NO 2 and NOx, PM2.5, PM10, EC, OC, diesel PM2.5, total PAHs, biomass burning PM2.5, and ammonium nitrate. The co-pollutants were also associated with increased risks of preterm birth. | Not determined. |
Estarlich et al. 2016 | Prospective cohort study of 2 409 pregnant women on the risk of preterm birth. Benzene and NO2were both measured in the study. | Benzene concentrations were measured outdoors in residential areas using passive samplers for 7 days. The median benzene concentration was 1.3 μg/m3 for the four regions | OR for a 1 μg/m3 increase in benzene were above 1 for scenarios evaluated in the one- and two-pollutant models for first trimester, second trimester, third trimester and entire pregnancy. The highest ORs were obtained for the entire pregnancy and the third trimester for women who spent more than 15 hours a day at home (OR: 1.59 in the one-pollutant model). Using the two-pollutant model adjusted for NO2, smoking, maternal age, maternal pregnancy weight, maternal and paternal height, gestational weight gain, and other factors, the authors reported an OR of 1.45 (95% CI, 1.00–2.09) per 1 μg/m3 (0.3 ppb) increase in benzene exposure in the third trimester among pregnant women who had spent more than 15 hours per day at home during their third trimester. | LOAEC for preterm birth = 1 μg/m3 increase in residential outdoor air |
Williams et al. 2019 | Cross-sectional study of rates of gestational diabetes mellitus (GDM) in 220 605 deliveries. Ethnicity was included in the analysis. Study included white (n = 109 396), Black (n = 49 093), Hispanic (n = 38 241) and Asian/Pacific Islander (n = 9068) participants. Thirteen other VOCs were evaluated besides benzene. | Participants were exposed to outdoor benzene at 15 hospital referral regions. Mean concentration was estimated 3 months before conception and during the first trimester of pregnancy: 0.24 ppb (0.77 μg/m3). | The authors observed that a preconception benzene exposure of 1.1 μg/m3 (0.34 ppb) or higher was associated with 25% increased odds (99% CI, 1.08–1.43) of gestational diabetes mellitus among white people and 41% increased odds (99% CI, 1.12–1.77) among Asian Pacific Islanders. They also observed that exposure to 1.1 μg/m3 (0.34 ppb) or more benzene during the first trimester of pregnancy was associated with 29% increased odds (99% CI, 1.04–1.59) of gestational diabetes mellitus among Asian Pacific Islanders. Similar effects were not observed among Black and Hispanic groups. Models were adjusted for maternal physical characteristics, parity, marital status and socioeconomic factors, but not co-pollutants. | LOAEC for increased risk of GDM = benzene concentration greater than 1.06 μg/m3 as an outdoor air concentration for a health region. |
Study | Participants | Exposure | Results | NOAEC/ LOAEC |
---|---|---|---|---|
Smith 2010 | Literature review on the effects of benzene on benzene-exposed workers and the general public. | Occupational exposures to industrial solvents, public exposure to manufacturing plants, emissions from fires, occupational environment, smoking | Cohort of rubber industry workers from the 1977 Pliofilm study revealed the leukemogenic effects of benzene exposure. Can also cause acute leukemia and potentially other hematological cancers. More evidence for the association of childhood leukemia and benzene exposure is emerging. Benzene exposure can affect the leukemogenic process through a multimodal mechanism. Specific SNPs (single-nucleotide polymorphism) in candidate genes result in susceptibility to benzene hematotoxicity. GWAS will be needed for further examination of genetic variation in benzene susceptibility. Benzene affects hematopoiesis at low levels of occupational exposure. No safe threshold of benzene exposure has been identified. | Not determined. |
Lan et al. 2004 | Cross-sectional study of 250 benzene-exposed shoe workers and 140 unexposed workers (age/sex matched) in clothing manufacturing facilities. 2/3 female; mean age 29.9 ±8.4 years; employed 6.1± 2.9 years | Individual benzene and toluene exposure was monitored for up to 16 months before phlebotomy and post-shift urine samples were taken. Workers were categorized into four groups by mean benzene levels measured during the month: controls, <1 ppm, 1 to <10 ppm, and ≥ 10 ppm. More than 100 of the exposed workers had exposures below 1 ppm. | All types of WBCs and platelets decreased in the < 1 ppm group and significant reductions were seen for CD4+ T cells, CD4/CD8+ ratio and B cells. Hemoglobin levels declined in workers exposed to >10 ppm. Adjustment for confounders (age, sex, current smoking, current alcohol drinking, BMI, recent infections, and log-transformed toluene air level when appropriate) did not significantly change the strength of associations. Hematological effects were observed for all concentrations of exposures (<10 ppm, <1 ppm over a previous year, and <40 ppm years cumulative exposure (p <0.05)). The same effect was observed in the group exposed primarily to benzene (<1 ppm). Significant dose-dependent decreases in colony formation from progenitor cells was observed. Greater proportional decrease in colony formation than in levels of differentiated WBCs and granulocytes, suggesting early progenitor cells are more sensitive than mature cells to the hematotoxic effects of benzene. SNPs in CYP2E1, MPO, and NQ01 genes were examined. Genotypes MPO 463GG and NQ01 465CT showed decreased WBCs and had a greater effect with exposure to benzene as seen in the exposed group. | LOAEC= <1 ppm or <40 ppm-years |
Bassig et al. 2016 | Cross-sectional study of 250 workers (164 females and 86 males) exposed to benzene in two shoe-manufacturing factories. Control participants consisted of 140 individuals working in other factories who were not exposed to benzene. | Initially benzene was measured through personal samples (passive monitoring badge) over one work shift for up to 16 months before blood sampling. Urine samples were collected post shift. Median benzene concentration for exposed workers was 1.2 ppm (3 800 μg/m3). Unexposed workers were not measured for benzene. Exposed workers were classified as: < 1 ppm (3 200 μg/m3); 1 to 10 ppm (3 200 to 32 000 μg/m3); and >10 ppm (32 000 μg/m3) | Compared to the controls, the workers exposed to benzene had significantly reduced WBCs (p trend <0.001), granulocytes (p trend <0.001), platelets (p trend = 0.001) and lymphocytes (p trend <0.01). Exposed workers had significant decreases for CD4 T cell counts (p trend <0.05) CD4:CD8 ratio (p trend <0.05), and B-cells (p trend <0.001). Chromosomal loss (as mean frequency of monosomy 7) was increased in benzene-exposed workers (p trend = 0.003). Markers of B-cell activation (soluble CD27 but not CD30) were significantly decreased in workers exposed to benzene (p trend = 0.05). No other co-pollutants were evaluated. Models were adjusted for age, sex, current smoking/alcohol consumption, BMI (body mass index), and recent infection. | Not determined. |
Harati et al. 2017 | Cross-sectional study of painters at an automobile manufacturing facility: 40 exposed and 40 unexposed. The exposure period ranged from 2 to 16 years (mean of 6.9 years). | Benzene was measured at the workplace of 20 exposed painters (3-hour samples). Average indoor concentration of benzene was 0.775 ppm (2 480 μg/m3) for painters. The air of the unexposed painters was not measured. | The group of painters exposed to benzene showed significantly increased MCHC (p <0.01) and significantly decreased eosinophil count (p <0.001). Other hematological parameters were affected but showed no statistical significance. No regression/analysis was done to distinguish the effects of benzene from other solvents. Models were adjusted for age, work experience, smoking and body mass index (BMI). Authors concluded that painters showed hematological effects at concentrations of less than 1 ppm. | LOAEC: 2 480 μg/m3 in workplace air (lack of measurement of benzene in unexposed painters' workspace). |
Zhang et al. 2016 | Cross-sectional study of 317 workers (144 female: 173 male) from 3 shoe factories. A total of 102 unexposed workers from office settings in banks and schools (52 females and 50 males) were included. | Measurement of benzene in the workplace was conducted through 15-minute samples collected 3 times a day in 3 different areas (sewing, molding and packing). Median benzene concentration was 1.57 ppm (5 024 μg/m3) at sewing, 2.60 ppm (8 320 μg/m3) at molding; and 1.79 ppm (5 278 μg/m3) at packing. Cumulative exposure of the workers was calculated in μg/m3-years by accounting for the time spent in each area and the years of employment with the specific median exposure levels/groups. The specific exposure groups include 0 ppm-years; 3.55 ppm-years (11 400 μg/m3-years); 6.51 ppm-years (20 800 μg/m3-years); 10.72 ppm-years (34 300 μg/m3-years); 20.02 ppm-years (64 100 μg/m3-years); and 40.71 ppm-years (130 200 μg/m3-years) | Significant increases in MN frequency were found in all exposure groups (p <0.001). Mean WBC counts were lower in exposed workers as a whole, compared to the unexposed workers, and statistically significant decreases were reported in the three highest exposure groups. Trend testing indicated a significant dose-response for the younger, older, and age-pooled groups. Models were adjusted for age, sex, smoking and drinking. No other pollutants were evaluated. Using benchmark dose software (BMD) a BMD and BMDL (Bench Mark Dose Level) were estimated for 5% and 10 % effect but also critical effects. The most sensitive BMDL was 0.21 ppm-years (627 μg/m3-years) for reduced MN frequency in exposed workers over 30 years. The BMDL (95% lower confidence limit) was 1.6 x 10 -5 ppm-years (0.0528 μg/m3-years). When critical effects and a Hill model were used instead of the 5% and 10% response rate, the most sensitive BMD for MN frequency was identified, 1.85 ppm-years (5 920 μg/m3-years) (BMDL was 0.22 ppm-years or 704 μg/m3-years). If these BMDLs are used, the TLV (threshold level values) would range from 0.0000125 ppm (0.04 μg/m3) to 0.053 ppm (170 μg/m3). |
Hematotoxic effects were associated with a BMCL ranging from 110 to 950 μg/m3 (34 to 300 ppb) benzene. |
Chen, Sun et al. 2019 | Cross-sectional study of 421 elderly residents from Nanjing, China. The exposure group comprised of participants that lived near a petrochemical complex (n = 240) and the control group resided in uncontaminated areas (n = 181). The age range of the participants was 50–71 years old. | Benzene exposure was measured in blood concentration along with toluene, ethylbenzene, m/p-xylene and o-xylene. | Higher blood BTEX concentrations were observed in the elderly group located near the petrochemical complex compared to those who were located elsewhere. Mean concentrations of BTEX were 1.2 to 6.7 times higher in elderly residents located near a petrochemical complex compared to residents living elsewhere. Significant differences for hematological parameters were observed between the exposed and unexposed group. Platelet counts and MCHC (mean corpuscular hemoglobin concentrations) were inversely associated with ln-transformed blood benzene concentrations. | Not determined. |
Doherty et al. 2017 | Prospective cohort study on residents of the US Gulf Coast who may have been occupationally exposed to benzene and control participants not exposed. 406 participants included were 21 years or older, previously participated in the Gulf Coast study. People who do not smoke, women, and clean-up workers were oversampled in the experimental population. | Benzene exposure (measured using blood benzene concentration) was occupational (oil spill clean-up workers) or through tobacco smoking. | Inverse associations were seen between blood benzene concentrations and hemoglobin concentrations and MCHC. Positive associations were seen for red blood cell distribution width and participants not exposed to tobacco smoke (n = 146). In participants exposed to tobacco smoke (n = 247) positive associations were seen between blood VOC concentrations and other hematological measurements (WBC counts, platelet counts). Associations were stronger for benzene than other co-pollutants. | Not determined. |
Vaughan Watson et al. 2021 | Longitudinal representative- cohort study of the US from the NHANES Cycles from 2005 to 2010. Participants were 12 years or older and had lab results in the VOC samples (n = 9203). Some participants were excluded from the study due to pregnancy, treatment of anemia, usage of chewing tobacco and smokeless tobacco products (n = 642). | Personal exposure to VOCs of participants in the NHANES Cycles from 2005 to 2010. Personal exposure levels were measured though blood samples. | Cox regression analysis was used to determine the association between complete blood count (CBC) measurements and specific blood VOCs. Individuals exposed to tobacco smoke had higher VOC levels than the unexposed group. Statistically significant associations were seen in the tobacco smoke group for BTEXS (Benzene, Toluene, Ethylbenzene, Xylenes, and Styrene), VOCs and hematological parameters, white blood cell count, hematocrit and hemoglobin (p values <0.0001 to 0.016). In the group not exposed to tobacco smoke, statistically significant associations between BTEX and platelet count were observed (p value <0.0001 to 0.02). The strongest association was with benzene for both the tobacco exposed and unexposed groups. | Not determined. |
Cakmak et al. 2020 | Cross-sectional study including 3 950 Canadian (provinces only) participants (ages 12 to 79) from CHMS Cycles 3 and 4 (2012–2015). | Personal exposure to VOCs (including benzene) collected through CHMS Cycles 3-4 from 2012–2015. Exposure measured by blood VOC concentration. | Associations for increased VOC (benzene included) blood concentration was reported with a 0.68% (95% CI 0.36, 1.0) to 0.91% (95% CI 0.52, 1.3) increase in hemoglobin and a 1.79% (95% CI 0.96, 2.62) to 4.11% (95% CI 3.11, 5.11) increase in WBCs. Benzene concentrations resulted in a hemoglobin increase of 0.89% (95% CI 0.47, 1.3). Increasing concentrations of VOCs resulted in increases in WBCs; strongest association was xylenes (unadjusted for benzenes) and resulted in a decrease in creatinine. Increase in benzene blood concentration resulted in a decrease of GGT (gamma-glutamyl transferase) for males (-4.74%, 95% CI -9.31, -0.17). For females, an increase in GM concentration of benzene resulted inan 8.02% increase in GGT (95% CI 4.03, 12.01). Smokers had a higher increase in WBCs compared to non-smokers (8.97%, 95% CI 5.29, 12.65, versus 1.64%, 95% CI − 0.02, 3.3). | Not determined. |
Study | Participants | Exposure | Results | NOAEC/ LOAEC |
---|---|---|---|---|
Berr et al. 2010 | Cross-sectional study of 5242 participants aged 55-65 from the GAZEL cohort. Other co-pollutants were evaluated including total petroleum solvents, total chlorinated solvents, and non-benzene aromatic solvents. | Exposure to benzene was occupational and based on the job exposure matrix. Median exposure was 11.9 ppm-years. Participants were classified into 3 different groups unexposed, moderate exposure (<median), and high exposure (≥median). | Analysis by logistic regression showed there was a greater risk of poor cognitive performance (DSST score <25th percentile) for those with high exposure to benzene and (OR = 1.58; 95% CI 1.31–1.90) other pollutants. (Models were adjusted for sociodemographic characteristics). Similar results were seen with Mini Mental State Examination (MMSE) for moderate (OR = 1.28 [1.03–1.59]) and high exposure to benzene (OR = 1.39, 95% CI 1.12–1.74). | Not determined. |
Sabbath et al. 2014 | Cohort study of 2 143 retirees from the GAZEL cohort (for cognitive testing). Lifetime exposure to chlorinated solvents, petroleum solvents and benzene were assessed using a job exposure matrix. | Benzene exposure was estimated by job titles and year(s) from ongoing industrial hygiene monitoring of employee workstations, and occupational medical data controls were matched to similar positions. Annual estimates for solvents were calculated. 26% of participants never exposed to benzene; 13% moderately exposed, 13% highly exposed. 17% last exposed before 1980 (among exposed), 9% exposed since 1980. | Exposure to high concentrations of solvents containing benzene is associated with poor cognition. Those retired with past high exposure throughout their lifetime were at the greatest risk and were exposed to solvents 12 to 30 years before testing. Moderate risk was observed for those with high exposure within the last 31 to 50 years. Individuals with recent high exposure showed signs of impairment in all domains and in domains not typically associated with exposure. General cognition was significantly associated with high lifetime exposure to benzene (RR = 1.7; 95% CI 1.06–1.31); a second test of general cognition showed an increased risk with high benzene exposure (RR = 1.31; 95% CI 1.11–1.56); also, high exposure was associated with decreased semantic fluency (1.25 (1.07–1.47). The most hazardous category, high proximal exposure, was significantly associated with increased risk across all solvent types. | Not determined. |
Sabbath et al. 2012 | Prospective cohort study of 4 134 gas and electric utility employees (mean age of 59). Other co-pollutants were evaluated including total petroleum solvents, total chlorinated solvents, and non-benzene aromatic solvents. | Benzene exposure was occupational and was estimated using a job exposure matrix. Employees were classified as: not exposed (n = 2882); low exposed (n = 626) and high exposed (n = 626). | High benzene concentrations were associated with a RR of 1.24 with a significant linear trend (p <0.05) for workers who were less educated. No dose-response relationship was seen in workers with higher levels of education. No other analyses were done to distinguish benzene effects from other co-pollutants. | Not determined. |
Kicinski et al. 2016 | Cross-sectional study of 895 ninth grade students. | Benzene concentrations were measured by urine samples for benzene metabolite t,t-MA | A 10-fold increase in urinary t,t-MA was associated with significantly decreased sustained attention (p = 0.02); diminished short-term memory (p = 0.001); higher mean reaction time (p = 0.001); and more errors of omission (p = 0.028). No other pollutants were evaluated. Adjustments were made for blood, parental education, gender, passive smoking, ethnicity, urinary creatinine, time of day and exam day. | Not determined. |
Kim et al. 2017 | Case-control study of 11 children with recurrent otitis media who visited Seoul National University Hospital from Nov 2014 to June 2015. Controls were children who had not had otitis media within the last year (n = 39). | Benzene concentrations were measured by urine samples for benzene metabolite, trans, trans-muconic acid (t,t-MA) | In the group of children with otitis media, passive smoking was significantly more common than the controls (p < 0.001). Concentrations oft,t-MA were significantly higher in the otitis media group (126.33 mg/g Cr) than the controls (52.661 mg/g Cr; p = 0.003). Other metabolites did not have a significant association with otitis media. | Not determined. |
Staudt et al. 2019 | Cross-sectional study of 1 085 to 2 471 individuals aged 20 to 59 from the years of the 1999 to 2004 NHANES Cycle. Study was a secondary analysis of NHANES data. Study examined associations of individual organic solvent exposures by measuring blood biomarkers for VOCs (benzene included) and with self-reported hearing loss, audiometrically assessed hearing loss and self-reported tinnitus. | Benzene and other VOCs were measured in blood. Median blood concentration of benzene was (0.04 ng/mL [IQR = 0.02, 0.11] vs. 0.03 ng/mL [IQR = 0.02, 0.09], p <0.001), the median varies for those who self-reported hearing loss and those who did not. The median blood concentration of benzene (0.06 ng/mL [IQR = 0.03, 0.11] vs. 0.03 ng/mL [IQR = 0.02, 0.09], p= 0.023) varied for those with audiometrically assessed hearing loss as compared to those without. | Benzene (OR = 1.43, 95% CI 1.15–1.78) and other VOCs showed a statistically significant association with increased adjusted odds of high-frequency hearing loss. No statistically significant association was seen between VOCs and occupational noise on high-frequency hearing loss. No association with tinnitus. | LOAEC= 0.04 ng/mL (in blood) |
Amin et al. 2018 | Cross-sectional study of 86 children ages 6 to 18. | Benzene concentrations in urine samples were used to measure a benzene metabolite,t,t-MA. | Adjustments were made for sex, age and passive smoking. The adjusted results showed statistically significant associations between t,t-MA and insulin resistance (p = 0.002), fasting blood glucose (p = 0.02) and fasting blood insulin (p = 0.001). At intermediate and low levels of benzene (t,t-MA), there were significant increases in superoxide dismutase (4.49-fold; p = 0.01) and malondialdehyde (3.54-fold; p = 0.034). | Not determined. |
Choi et al. 2014 | Cross-sectional study of 505 participants aged 60+ from Seongbuk-gu Community Center in Seoul, South Korea. Participants were followed from August 2008 to August 2010. | Benzene exposure was measured using the urinary metabolitet,t-MA. The homeostatic model assessment index (HOMA-IR) was also measured in participants (insulin resistance). Air exposure to benzene was measured in 63 participants through a passive diffusion-sampling device for 10 days. The exposure concentration to benzene in the air was < 0.0002 μg/m3. | In participants, dose-dependent concentrations oft,t-MA increased levels of insulin resistance (p trend <0.001) and oxidative stress from urinary malondialdehyde (p trend <0.001). The upper quartiles oft,t-MA concentrations in participants had associations with increased insulin resistance [Q3: 3.33 (95% CI: 1.90–5.84); Q4: 2.07 (95% CI: 1.02–4.22)]. | Not determined. |
Study | Participants | Exposure | Results | NOAEC/ LOAEC |
---|---|---|---|---|
Scholten et al. 2020 | Systematic review of 16 CA studies (1 356 participants) and 13 MN studies (2 097 participants). A meta-analysis was conducted to estimate the exposure-response relationships between occupational benzene exposure and its cytogenetic effects on CA and MN. Benzene had to be the main pollutant in order for studies to be included in the analysis. | Systematic reviews of CA and MN included studies with sufficient quantitative information on benzene exposure. | Articles that had benzene as the main pollutant and
had sufficient data outcomes were used in the dataset
for statistical analysis. All CA studies combined
showed a 0.27% increase in CA (95% CI: 0.08%, 0.47%) per
ppm benzene exposure. The MN studies combined showed
an increase of 0.27% MN (95% CI: -0:23%, 0.76%) per ppm benzene exposure. The authors observed considerable between-study heterogeneity for both endpoints (I 2 > 90%). |
Not determined. |
Angelini et al. 2016 | Systematic review and meta-analysis of occupational populations exposed to petroleum/petroleum derivatives and alterations in MN frequency. Used 34 datasets from 26 papers. | The exposures varied in each study. The main focus was occupational exposure, with most benzene exposure levels greater than 100 μg/m3. Some studies reported benzene concentrations of about of 10 μg/m3. | Occupational workers exposed to benzene, toluene and/or xylenes had a 1.38-fold greater MN frequency in peripheral blood lymphocytes than unexposed workers. Twenty-three of the datasets were found to have an increase in MN frequency. The summary mean difference in MN frequency between exposed and unexposed individuals was 1.64 (95% CI: 0.80–2.47). | Not determined. |
Ayi-Fanou et al. 2011 | Cross-sectional study of 57 people exposed to urban air (taxi-motorbike drivers, street food vendors and gasoline salesmen) versus those living in rural (17 people) and suburban areas (20 people). While individuals who said they smoked were not included in the study, people who use wood fires for meals or consume smoked fish may have been. Most of the participants were men (88%) and the average age was 30. | Average benzene concentrations measured in outdoor air were reported to be 76.0 μg/m3 (in the city), 4.3 μg/m3 (suburban) and 3.4 μg/m3 (rural). Measurements of PAHs (polycyclic aromatic hydrocarbons) were taken outdoors. The level of total PAHs in urban air was 103 μg/m3 while the levels in suburban and rural areas were 2.5 and 1.6 μg/m3, respectively. | Elevated levels of DNA adducts were found in urban participants (30 adducts per 108 nucleotides) than suburban or rural participants (in the range of 2 to 3 adducts per 108 nucleotides). Regression or other analysis was not completed to distinguish the effects of benzene from other co-pollutants. | Not determined. |
Peluso et al. 2013 | Cross-sectional study of 148 schoolchildren (aged 6-14 years) from an urban school (Sarroch, n = 75) and a rural school as a control (Burcei, n = 73) (more females than males participated). Ethylbenzene was also evaluated. | Benzene was measured outside with diffusive samplers over a 3-week period in the school gardens in the city (Sarroch): residential areas, the centre, industrial areas, and the rural village (Burcei). A total of 33 samples were taken at 19 locations. Mean benzene concentrations were 1.4 μg/m3 at rural village school gardens. Other areas of Sarroch had a significantly higher mean benzene concentration with values ranging from 2.7 to 3.8 μg/m3 in all areas except near the industrial park (5.8 μg/m3). | Children from Sarroch had significantly higher malondialdehyde–deoxyguanosine adducts than children in the rural village (average of 2.53 times greater; p<0.001). Additionally, children from Sarroch had significantly higher levels of bulky DNA adducts compared to the rural village children (average of 1.90 times greater; p= 0.003). Models were adjusted for age, gender and residence. No other analyses were completed to distinguish the effects of other co-pollutants from benzene. | The LOAEC seems to be in the range of 2.7 to 5.8 μg/m3. |
Li et al. 2017 | Cross-sectional study of 96 petrochemical industry workers (65 male: 31 female), 100 office worker controls were matched. All petrochemical industry workers were from the same plant. Toluene was also evaluated. | There were 36 sampling sites in the petrochemical plant. The median benzene concentration indoors was 110 μg/m3 for benzene-exposed workers and < 10 μg/m3 for unexposed office workers. Urinary SPMA was measured as an indicator of exposure (median urinary SPMA was 2.6-fold higher in benzene-exposed workers) (p<0.001). | The hematological parameters (blood cell counts of leukocytes, neutrophils, lymphocytes and monocytes) measured in exposed workers did not differ significantly from the values for controls. Genetic damage (% tail DNA) was 11% higher for exposed workers (p = 0.012). Levels of urinary SPMA were found to be inversely associated with MGMT methylation (mean SPMA levels were 0.00176 mg/g Cr for workers and 0.00068 mg/g Cr for controls). Models were adjusted for age, sex, body mass index (BMI) and blood cell counts. | LOAEC = 110 μg/m3 for DNA damage. |
Ruchirawat et al. 2010 | Cross-sectional study of non-smoking adults (ages 18-45) employed at petrochemical laboratories (n = 31), gasoline service stations (n = 31) and a mail sorting site (n = 34). Participants were gender matched. The other pollutants evaluated were benzo[a]pyrene and 1,3-butadiene. In the second part of the study, children in the city (n = 76–128) and in rural areas (n = 55-69) were evaluated. The third part of the study examined workers at temples where incense was burned (n = 35–41) and control office workers where incense was not burned (n = 23–35). |
Benzene was sampled passively in the various workplaces. The mean benzene concentration was 2.34 μg/m3 in the control workplace, 85.26 μg/m3 at the petrochemical factories and 207.94 μg/m3 at the gasoline service stations. In the second part of the study, benzene concentrations were measured in rural (control) and urban school areas. Mean benzene concentrations were estimated to be 8.40 μg/m3 and 19.38 μg/m3 for rural and urban schoolchildren, respectively. In the third part of the study, benzene concentrations were measured at offices (control) and at temples. Mean benzene concentrations were estimated to be 26.49 μg/m3 and 45.90 μg/m3 for control workers and temple workers, respectively. |
In comparison to control workers, petrochemical workers had significantly increased signs of DNA strand breaks (increased Olive tail moment; p < 0.01) and significantly reduced DNA repair capacity (dicentrics and deletions per metaphase; p < 0.01). Gasoline attendants had significantly increased levels of DNA adducts (p < 0.001), DNA strand breaks (increased tail length Olive tail moment; p <0.001) and significantly reduced DNA repair capacity (dicentrics and deletions per metaphase; p <0.001). In the second part of the study, urban schoolchildren showed a significantly increased DNA adducts (p <0.001), DNA strand breaks (increased tail length Olive tail moment; p <0.001), and significantly reduced DNA repair capacity (dicentrics and deletions per metaphase; p <0.001) in comparison to rural schoolchildren. In the third part of the study, temple workers had significantly increased DNA adducts (p < 0.001), DNA strand breaks (increased tail length Olive tail moment; p <0.001) and significantly reduced DNA repair capacity (dicentrics and deletions per metaphase; p <0.01 to p <0.001) compared to controls. No other analyses were done to distinguish the effects of benzene from other co-pollutants. |
LOAEC for DNA changes = 85.26 to 207.94 μg/m3 in workplace air. |
Bassig et al. 2016 | Cross-sectional study of 250 benzene- exposed workers (164 females: 86 males) from shoe manufacturing factories. It included 140 control unexposed workers (88 females: 53 males) from other factories. | Initially benzene was measured through personal samples (passive monitoring badge) over 1 work shift for up to 16 months before blood sampling. Urine samples were collected post shift. Median benzene concentration for exposed workers was 1.2 ppm (3 800 μg/m3). Unexposed workers were not measured for benzene exposure. Exposed workers were classified as follows: <1 ppm (3 200 μg/m3); 1 to 10 ppm (3 200 to 32 000 μg/m3); and >10 ppm (32 000 μg/m3) | Compared to the controls, the workers exposed to benzene had significantly reduced white blood cells (p trend < 0.001), granulocytes (p trend <0.001), platelets (p trend = 0.001) and lymphocytes (p trend <0.01). Exposed workers had significant decreases in CD4 T cell counts (p trend <0.05), CD4:CD8 ratio (p trend <0.05), and B-cells (ptrend <0.001). Chromosomal loss (as mean frequency of monosomy 7) was increased in benzene-exposed workers (ptrend = 0.003). Markers of B-cell activation (soluble CD27 but not CD30) were significantly decreased in workers exposed to benzene (p trend = 0.05). No other co-pollutants were evaluated. Adjustments were made for age, sex, current smoking, current alcohol consumption, BMI, and/or recent infection. | Not determined. |
Ji et al. 2012 | Cross-sectional study of 33 male workers exposed to benzene in factories and 33 workers not exposed from 3 Chinese factories in Tianjin, China. | Personal measurements of benzene exposure were conducted using passive air badge monitors for an 8-hour workday and post shift urine samples. A month later, participants had their personal exposure levels measured with the passive air badge and a urine sample. A fasting blood sample was also collected. Benzene concentrations of the exposed workers were <24 ppm. | In-situ hybridization allowed for examination of chromosomal abnormalities on sex chromosomes and chromosome 21 due to benzene exposure. Chromosomal aneuploidies were observed in blood lymphocytes and sperm cells. In blood lymphocytes, chromosomal additions were seen on chromosome 21 but not on sex chromosomes. In sperm, the sex chromosomes gained additional chromosomes, chromosome 21 remained diploid. | Not determined. |
Zhang et al. 2012 | Cross-sectional study of 20 exposed females and 8 exposed males from a larger study of 250 workers at 2 shoe factories. An additional 14 control participants selected from the larger cross-sectional study including 140 unexposed controls. | Benzene concentrations were measured in the workplace one month before blood collection. Mean benzene concentrations were as follows: controls were 0.04 ppm (128 μg/m3); low exposure = 2.64 ppm (8 448 μg/m3); and high exposure = 24.19 ppm (77 408 μg/m3). | An increase in monosomy (an indicator of chromosomal loss) was found in workers exposed to a low benzene concentration (20–28% increase at 8 448 μg/m3; p = 0.0056)) and a high benzene concentration (32–48% increase at 77 408 μg/m3; p = 0.0045) in CFU-GM cells (blood progenitor cells). WBCs were significantly reduced in the high exposure group relative to the controls (p <0.001); however, the p-trend for decreased WBCs across all concentrations was significant (p trend = 0.0027). No other pollutants were evaluated. | LOAEC for chromosomal loss = 8 448 μg/m3 in workplace air. |
Hoxha et al. 2009 | Cross-sectional study of 77 traffic officers exposed to benzene (30 females: 47 males) and unexposed indoor office workers (19 females: 38 males). Toluene was also measured. | Benzene concentration was measured outdoors through personal badge sampling over an entire work shift. Mean benzene concentration was 31.8 μg/m3 for traffic officers and 13.0 μg/m3 for unexposed workers. In the exposed traffic officer group, benzene exposures were further grouped into low traffic intensity (n = 32; mean = 26.3 μg/m3) and high traffic intensity (n = 45; mean = 35.7 μg/m3). | Compared to the controls, exposed traffic officers had significantly reduced relative telomere length (p <0.001). In the traffic officer group: high traffic intensity groups were found to have significantly reduced telomere length as compared to the low traffic intensity group (p <0.001). For all exposures, the plotted decline of leukocyte telomere length with increasing benzene concentrations had a p value of 0.004, toluene, a p value of 0.008. Adjustments made for age, gender, smoking, and pack-years. No other analyses were done to distinguish the effects of benzene from those of other co-pollutants. | LOAEC for reduced leukocyte telomere length = 31.8 μg/m3 |
Bassig et al. 2014 | Cross-sectional study of 43 benzene- exposed workers (21 females: 22 males) from 3 factories, 43 control workers (21 females: 22 males) (age and gender matched) from 2 factories that did not use benzene. Exposed workers worked 6 months in the factories. Other substances measured include toluene, ethylbenzene, and xylenes (TEX). | Benzene indoor concentrations were measured for exposed workers and a subset of controls using passive dosimetry badges. In exposed workers, the mean benzene concentration was 62.7 ppm (200 000 μg/m3). Participants were further subdivided into a lower exposed group (21 workers) with a mean benzene concentration of 14.0 ppm (44 800 μg/m3) and a high exposed group (n = 22) with a mean benzene concentration of 109.2 ppm (349 400 μg/m3). | In comparison to controls, telomere length was significantly increased in workers exposed to concentration greater than 31 ppm (99 200 μg/m3) (p = 0.03). No significant effect was found in workers at lower concentrations. However, considering all exposures, a p trend value of 0.05 was found for altered telomere length in workers exposed to benzene. Models were adjusted for age, sex, recent smoking and body mass index (BMI). Regression or other analyses were not done to distinguish benzene effects from those of other co-pollutants. | The NOAEC for altered telomere length = 44 800 μg/m3; the LOAEC for altered telomere length = 99 200 μg/m3 as an indoor workplace concentration. |
Uzma et al. 2010 | Cross-sectional study on 428 benzene-exposed gasoline filling workers and 70 control (non-exposed) workers. It was conducted in Hyderabad, Andhra Pradesh, India. | The exposed workers were monitored for benzene exposure using organic vapor passive dosimetry badges which they wore during their work shift. Participants also gave 2 samples of blood and urine pre-work shift and post-work shift. | An increase in concentrations of benzene was found in the exposed workers relative to the unexposed workers. Increases in reactive oxygen species and malondialdehyde were seen in exposed workers. A decrease in concentrations of glutathione and total superoxide dismutase were also seen in exposed workers in comparison to the controls. Statistically significant decreases in levels of CD4 T cells, immunoglobulin and the CD4:CD8 ratio were obtained for exposed workers in comparison to control workers. Overall, p53 expression was higher in the exposed workers than in the control workers. | Not determined. |
Carugno et al. 2012 | Cross-sectional study of 519 adult participants including occupationally exposed individuals (153 bus drivers in Genoa; 78 gas station attendants and 77 police officers in Milan; 33 petrochemical plant workers in Cagliari). Benzene was the only substance evaluated. | Personal benzene concentrations were monitored using passive badge samplers for 5 to 6 hours. Only benzene was measured. GM concentrations were as follows: bus drivers = 20.5 μg/m3; police officers = 25.0 μg/m3; gas station attendants = 69.9 μg/m3; petrochemical workers = 35.4 μg/m3. The GM range for referents was 5.9 μg/m3 (distant Cagliari) to 8.7 μg/m3 (Genoa). | In the 3 cities, benzene-exposed participants had a higher mtDNAcn (mitochondrial DNA copy number) than the corresponding control participants. In Genoa, bus drivers had 0.90 relative units vs. 0.75 for referents (p = 0.019); In Milan, gas attendants had 0.90 relative units and police officers, 1.10, vs. 0.75 in referents (p-trend = 0.008); in Cagliari, petrochemical workers had 1.63 relative units vs. 1.25 for referents living close to plants and 0.90 for referents living farther away (p-trend = 0.046). Data from all 3 cities were combined, and it was estimated that the IQR increase in benzene was associated with a percent increase of 10.3% in mtDNAcn (p <0.001). An association was observed between mtDNAcn and leukemia related epigenetic markers LINE-1 hypomethylation (-2.41%; p = 0.007) and p15 hypermethylation (+15.95%, p = 0.008). No other analyses were performed to distinguish benzene effects from the effects of other co-pollutants. | Not determined. |
Study | Participants | Exposure | Results | NOAEC/ LOAEC |
---|---|---|---|---|
Rinsky et al. 1981 | Retrospective cohort study of exposed workers in a rubber hydrochloride manufacturing plant. Study examining leukemia mortality in exposed workers. Exposed participants were divided into two groups, group 1 (n = 748) and group 2 (n = 258). Unexposed (n = 177) workers were the controls for this study. The study covered the period from 1940 to 1975. | Benzene exposure concentration was determined from the job titles held by participants and reports of benzene concentration during the rubber hydrochloride manufacturing (obtained from the Industrial Commission of Ohio, Health Department of Ohio, University of North Carolina, NIOSH and company monitoring). From an analysis of all the exposure data, it was concluded that benzene exposures in each year fell within the national recommended 8-hour time-weighted averages (TWA). TWA exposures for the following years include the following: 1941-100 ppm, 1947- 50 ppm, 1948- 35 ppm, 1957- 25 ppm, 1963-25 ppm, and 1969-10 ppm. | In group 1, workers were exposed to a benzene area for at least one day. There were 180 deaths in group 1. The expected number of deaths from group 1 was 161 (SMR = 111). The increase in deaths was not statistically significant but was unusual. Group 1 also showed a statistically significant number of deaths attributable to malignancies in the lymphatic and hematopoietic system: 10 observed, 3 expected (p <0.01). Seven leukemia cases were documented as opposed to 1.25 expected (p <0.001). In group 1, 58% of workers were exposed to benzene for less than a year. Group 2 had 49 deaths, with 1 death caused by leukemia against the expected of 0.46 (SMR = 217). Leukemia deaths in group 2 (n = 7) were associated with benzene exposure for more than 5 years, with an SMR of 2100. The cells of individuals with leukemia were myelogenous or monocytic. | Not determined. |
Rinsky et al. 2002 | Longitudinal cohort study of a follow-up of rubber hydrochloride manufacture workers from a 1981 cohort study by Rinsky et al. The study covered the period from 1981 to 1996. It originally included 1 165 white males and added 1 291 workers with no written indicators of race/gender. | Exposure to benzene occupationally in the plant was divided into four categories as a measurement of ppm-years. The four different categories included 1 ppm-day to 39.99 ppm-years; 40–199.99 ppm-years; 200–399 ppm-years; and 400 or more ppm-years. | For the entire cohort, the levels of malignancies (lymphatic and hematopoietic neoplasms) increased and the SMR was 1.64 (95% CI = 1.06–2.42). The SMR for leukemia for the entire cohort was 2.56 (95% CI = 1.43–4.22). In all, 15 of the deaths within the cohort were linked to cumulative exposure of at least 1 ppm-day. There were 8 multiple myeloma cases in the study. Cox proportional modelling showed that the duration of exposure had an increased relative risk estimate of 39.2% per year of exposure (p = 0.004). The average exposure rate had an increased risk of 7.6% per ppm of benzene (p = 0.048). The risk for multiple myeloma increased by an estimated 1.003 per ppm-year of exposure to benzene or 1.13 for 40 years of exposure to a concentration of 1 ppm (95% CI = 0.95-1.34) | Not determined. |
Yin et al. 1987 | Retrospective cohort study of 233 benzene factories and 83 control factories in 12 Chinese cities. In all, 28 460 benzene-exposed workers and 28 257 non-exposed workers were included. | Benzene exposure among participants with leukemia ranged from 10 to 1 000 mg/m3. Seven of the participants with leukemia had reported chronic benzene poisoning before their diagnosis. | In the group of workers exposed to benzene there was 30 cases of leukemia with 25 deaths, only 4 cases of leukemia were found in the unexposed group. Average age of exposure was 27.8 years and the average latency of leukemia was 11.4 years. The SMR for the benzene-exposed leukemia cases was 5.74 (p <0.01). Benzene-related leukemia incidence was highest among paint and rubber manufacturers. | Not determined. |
Yin et al. 1996 | Cohort study of benzene-exposed workers (n = 74 828) and unexposed workers (n = 35 805) from 1972 to 1987. This study was conducted in 672 benzene-exposed factories and 69 unexposed factories in 12 Chinese cities. | Benzene exposure was assessed based on job title, work unit and factory. Exposure was based on historical data from 1 427 work units and 3 179 job titles. Occupational benzene exposure ranged from 25 to33 ppm before 1975 and 8 to15 ppm after. Besides occupational exposure to benzene, urban and highly trafficked areas had a benzene concentration of 1 to150 mg/m3. | Participants were followed for an average of 10.5 to 11.7 years. RR (age adjusted) for cancer mortality of exposed workers vs. unexposed workers was 0.4 to 2.8 (women) and 0.9 to 3.3 (men). The RR for leukemia was 2.8 for women and 2.1 for men; the RR for lung cancer was 1.5 for men and 1.0 for women. For leukemia, MDS and lymphoma, the RR values were significantly elevated: 2.5 to 3.1 in exposed workers. | Not determined. |
Glass et al. 2003 | Case-control study of 79 Australian petroleum industry workers between 1981 and 1999. Controls (n = 395) were age matched. | Exposure levels of workers were determined individually for both cases and controls. Estimation of exposure included job history of the workers and the measured exposures of job-tasks in the petroleum industry. A task-based algorithm was used to formulate individual exposure levels that included individual measurement parameters such as job title, task-, site-, and era-specific data. Cumulative exposure was calculated. | An increase in exposure to benzene was not found to have an increase in NHL or multiple myeloma. ORs increased for leukemia for the 3 highest exposure groups compared to the lowest exposure group (≤ 1 ppm). The greatest exposure group had 7 of the 33 cases of leukemia. OR of the two highest exposure groups was 11.3 (95 % CI = 2.85– 45.1). Cumulative exposure as a continuous variable had an OR of 1.65 (95% CI = 1.25–2.17). No association was observed for leukemia and duration of employment or start date. Leukemia risk and exposure to benzene concentrate had a strong association. High exposure intensity had a strong association with leukemia risk, at increments of 0.8 to 1.66 ppm; those with exposure to high intensity of benzene were 20 times more likely to be diagnosed with leukemia. | Not determined. |
Glass et al. 2005 | Nested case-control study of a re-analysis of a study on benzene exposure and risk of leukemia. This study re-examined the Health Watch prospective cohort study of 18 000 employees who worked in the Australian petroleum industry for 5 years and the association of development of NHL and multiple myeloma. | Exposure levels were retrospectively estimated for each individual using an algorithm in a relational database. Exposure measurements were given by the Australian petroleum companies to estimate exposure for specific job tasks. Participants started work after 1965 and had an average exposure of 20 years. In all, 85% of the cumulative exposure measurements from participants were below 10 ppm-years and 3.6% had exposure levels above 40 ppm-years. Two exposure metrics for benzene exposure: Cumulative exposure (ppm-years): ≤ 2, >2–4, > 4–8, >8–16, >16 Intensity of highest exposed job (ppm) ever held, controlling for career duration: ≤ 0.1, >0.1–0.2, >0.2–0.4, >0.4–0.8, >0.8–1.6, > 1.6–3.2, >3.2 |
The re-analysis of the Health Watch study showed that the 7 leukemia case sets with cumulative exposure greater than 16 ppm-years the (95% CI) OR was 51.9 (6.6–477) when compared to the two lowest exposed categories combined (≤ 2 ppm-years). Same conclusion with or without high exposure events (HEEs). ORs increased for leukemia with cumulative exposure treated as a continuous variable OR 1.10 (1.04–1.16) per ppm-year. HEEs added to cumulative exposure the OR was lower 1.03 (1.01–1.05) per ppm-year. Controlling for exposure duration leukemia was associated with high intensity exposure (greater than 0.8 ppm). | LOAEC= 16 ppm-years or 0.8 ppm |
Hayes et al.1996 | Cohort study of 35 805 unexposed workers and 74 828 exposed workers from industrial manufacturers. Exposed workers worked from 1972 to 1986 in 672 factories in 12 Chinese cities. Unexposed workers worked from 1972 to 1987 in 40 factories. Adjustments were made for birth date, sex, and occupational data. | Benzene concentrations were determined by factory level exposure and the job title held by each participant. The average occupational exposure to benzene had 6 levels: <1 ppm, 1 <5 ppm, 5 <10 ppm, 10 <25 ppm, 25 <50 ppm, and 50+ ppm. These levels were estimated by reports from local industrial hygienists and industrial health personnel. | Mortality (all-causes) minimally increased with greater cumulative exposure to benzene (p-trend <0.05), and when adjusted for cancer deaths (p trend <0.01). Non-malignant deaths were not associated with cumulative benzene exposures. High cumulative exposure increased mortality of HLM and lung cancers (both p-trend = 0.01). Model adjustments were made for birth date, sex, and occupational data. | Not determined. |
Schnatter et al. 2012 | Pooled case-control study of 3 petroleum worker studies from the UK, Canada and Australia to explore the dose-response relationships of benzene concentration and lymphohematopoietic (LH) cancers. Five LH cancers were chosen for analysis: AML (60 case participants), CLL (80 case participants), CML (28 case participants), MDS (29 case participants) and MPD (30 case participants). Case participants were age matched to 4 or 5 controls that were free from LH cancers. | Benzene exposure was estimated by the job position from each participant. Measurements for each participant's cumulative exposure to benzene was determined by the maximum benzene exposure intensity and duration in each job years (ppm-years). Jobs were assessed qualitatively to determine the likelihood of exposure to benzene concentration >3 ppm for 1 to 60 minutes at least weekly. The probability of dermal exposure (none, low, medium or high) was also assessed. Median (25th–75th quartile) cumulative benzene exposure (ppm-years) among cases and controls: -AML: cases 1.2 (0.2–5.3); controls 0.6 (0.1–4.0) -CML: cases 1.7 (0.6–3.4); controls 0.9 (0.2–4.9) -CLL: cases 1.1 (0.2–4.4); controls 1.1 (0.2–4.5) -MDS: cases 3.4 (0.4–8.9); controls 1.4 (0.2–3.8) -MPD: cases 1.7 (0.3–5.0); controls 1.1 (0.2–4.0) Six exposure metrics were used to best estimate benzene exposure: 1) cumulative exposure (ppm-years); ref group: ≤0.348 ppm-years 2) duration of employment (years); ref group: ≤15.6 years 3) average exposure intensity (ppm); ref group: ≤0.016 ppm 4) maximum exposure intensity (ppm); ref group: ≤0.016 ppm 5) Peak exposure (at least 1-year employment in jobs likely experiencing >3 ppm exposure for 15 to 60 minutes at least weekly); ref group: not >3 ppm. 6) Dermal exposure (the highest job-specific relative probability of skin contact for at least a year). Ref group: no dermal exposure |
MDS association with cumulative benzene exposure showed a monotonic dose-response relationship (highest vs. lowest tertile, >2.93 vs. ≤ 0.348 ppm-years, OR = 4.33, 95% CI = 1.31–14.3). For peak benzene exposures (>3 ppm) the risk of MDS was increased to high and medium certainty diagnoses (peak exposure vs no peak exposure, OR = 6.32, 95% CI = 1.32–30.2). Minimal evidence was seen for dose-response relationships of AML, CLL, CML or MPD |
NOAEC for cumulative exposure: ≤0.348 ppm-years LOAEC for cumulative exposure: >2.93 ppm-years LOAEC for peak exposure: >3 ppm |
Rushton et al. 2014 | Pooled case-control study of three petroleum worker studies from the UK, Canada and Australia. Study included 60 cases of AML with 241 matched controls and 80 cases of CLL with 345 matched controls. | Occupational benzene exposures were quantitatively estimated by using work histories and era-specific data. Country-specific and era-specific data were used to determine shift-averaged estimates of benzene exposure and were adjusted for differences in the workplace. Six exposure metrics were derived: cumulative exposure (ppm-years); duration of employment (years); average exposure intensity (ppm); maximum exposure intensity (ppm); peak exposure (at least 1 year in job with >3 ppm exposure for 15 to 60 min at least weekly) (Yes/No); dermal exposure (the highest job-specific relative probability of skin contact for at least a year) (none, low, medium, high). Benzene exposures were low and mean average intensity for AML cases was 0.18 ppm and 0.20 ppm for controls. For CLL, the average intensity was 0.19 ppm for cases and 0.21 ppm for controls. |
Categorical analyses showed increased ORs for AML with several exposure metrics. There was no relationship between benzene exposure and CLL except for an increased risk in refinery workers in Australia. | Not determined. |
Linet et al. 2015 | Retrospective cohort study of workers exposed to benzene (n = 74 827) and unexposed workers. Workers were from factories that manufactured coatings, rubber, chemicals and shoes. | There was no attempt to quantify benzene exposure | Workers exposed to benzene had an increased risk of mortality for all causes (RR = 1.1); all neoplasms (RR = 1.3); respiratory diseases (RR = 1.7); diseases of blood forming organs (RR = infinite – due to none occurring in unexposed cohort); lung cancer (RR = 1.5). Increased incidence for MDS/AML (RR = 2.7), CML (RR = 2.5); all lymphoid disorders included excesses of NHL (RR = 3.9); all lymphoid leukemia (RR = 5.4) was observed in workers exposed to benzene. Adjustments were made for sex, age and calendar year. No other pollutant was evaluated. | Not determined. |
Stenehjem et al. 2015 | Case-cohort study of 24 917 oil workers, most of whom were exposed to benzene for less than 15 years. The study population consisted mainly of males and there were 112 cases of lymphohematopoietic cancer (LH). A reference cohort was included (n = 1 661) of workers without LH cancer. | Exposure was measured multiple times using different techniques. Parameters to determine estimates of benzene exposure were average intensity, cumulative exposure and peak exposure. Average intensity measurements had 3 categories; <0.007 ppm (<22 μg/m3); 0.007 to 0.013 ppm (22 to 42 μg/m3); and 0.013 to 0.040 ppm (42 to 130 μg/m3). Cumulative exposure measurements also had three categories: <0.037 ppm-years (120 μg/m3-years); 0.037 to 0.123 ppm-years (120 to 390 μg/m3-years); and 0.124 to 0.948 ppm-years (390 to 3,030 μg/m3-years). | In all three categories of benzene intensity and cumulative exposure hazard ratios almost had a near significant p trend; cumulative trend (p trend = 0.052), average intensity (p trend = 0.092) and average peak (p = 0.056). A significant association was seen for the hazard ratio of multiple myeloma and cumulative exposure (p trend = 0.024). A suggestive trend for CLL for average intensity was observed (p trend = 0.094). No other analysis was done to distinguish the effects of benzene from other co-pollutants. Adjustments were made for age, exposure to benzene from other jobs and smoking (daily). | Not determined. |
Wong et al. 2010 | Case-control study in hospital setting of 722 newly diagnosed cases of AML and 1444 gender and age matched controls from 29 hospitals. Other pollutants evaluated include TEX (toluene, ethylbenzene, and xylenes), diesel fuels, ethylbenzenes, xylenes and atc. | Benzene exposure was measured through questionnaire answers and job titles of participants. Maximum exposure categories were EG0 (non-exposure); EG1 (<1 000 μg/m3); EG2 (1 000 to 10,000 μg/m3); EG3 (10 000 to 100 000 μg/m3); and EG4 (>100 000 μg/m3) | Exposed workers compared to workers never exposed, the ORs were significantly increased above 1 for total AML and the AML subtypes; AML-RCA (OR = 1.61) and APL (OR = 1.95). ORs for total AML were the greatest when the length of the exposure was <10 years (OR = 1.99) but lower ORs were observed for 10 to 20 years of exposure (OR = 1.44) and >20 years (OR = 0.74). Maximum exposure for total AML had progressively higher ORs (highest OR of 2.05 in EG3 and EG4; p trend = 0.01). Delayed first exposure resulted in a higher OR value (OR = 4.18 when first exposure was after 2000 while ORs were lower when exposure was earlier). Multivariate analysis resulted in a non-significant OR of 1.20 for AML from benzene. | Not determined. |
Talibov et al. 2014 | A nested case-control study from the Nordic Occupational Cancer Study (NOCCA). The NOCCA study involved 15 332 cases of AML diagnosed in Finland, Norway, Sweden and Iceland during the years 1965 to 2005. Controls (n = 76 660) were matched to cases by year of birth, sex and country. | Using census records, occupational information was recorded from each country and linked to the Nordic Job Exposure Matrix for quantitative estimates of occupational exposure to benzene. Benzene exposure was determined by the proportion of those exposed (P) and the mean level of exposure (L) among exposed persons in a specific job and time period. Authors assumed the employment period started at 20 years old and ended at 65. Cumulative exposure to benzene was determined by variables P, L and employment period (T). Benzene cumulative exposure (CE) values were categorized as follows (ref: individuals with no exposure to benzene): Low exposure level (≤3.7 ppm): <50th percentile among the exposed cases and controls; 430 (3%) exposed cases and 1 999 (3%) exposed controls Moderate exposure level (3.7–13.6 ppm): between the 50th and 90th percentile inclusive; 310 (2%) exposed cases and 1 633 (2%) exposed controls High exposure level (>13.6 ppm): >90th percentile; 68 (0.4%) exposed cases and 418 (0.5%) exposed controls. |
Null associations between any level of cumulative exposure to benzene and AML. | Not determined. |
Ghazawi et al. 2019 | An ecological study of Canadian AML incidence and mortality from 1992 to 2010. Three population-based cancer databases were used: Canadian Cancer Registry (CCR), the Registre Québécois du Cancer (LRQC) and Canadian Vital Statistics (CVS). In the years 1992 to 2010, approximately 18 085 patients were diagnosed with AML. | Benzene exposure was not measured for individual cases. | AML incidence was highest at Canadian US border cities such as Sarnia, Thunder Bay, Sault Ste. Marie, St. Catharines and Hamilton. Incidence of AML in downtown Sarnia was 106.81 (95% CI, 70.96–161.86) cases per million, 3 times the Canadian average. Sarnia also has the most petrochemical plants in Canada. Sarnia has over 60 refineries and 40% of Canada's chemical industries. CAREX Canada's data on benzene exposure show an association between cities with high benzene exposure and high AML incidence and mortality. | Not determined. |
Glass et al. 2014 | A pooled case- control study of three petroleum worker studies from Canada, the UK and Australia to examine dose-response relationships between benzene exposure and lymphohematopoietic (LH) cancers. The focus of this paper is on MPD and CML separately. In all, 28 cases of CML and 122 matched controls were included; 30 MPD cases and 124 matched controls were also included. | Quantitative estimates for benzene exposure were determined for the job of each participant. These estimates were used to generate exposure estimates for each participant. Dermal exposure and peaks of exposure greater than 3 ppm of benzene for 15 to 60 minutes weekly were assessed. Mean average exposure intensity was 0.3 ppm for CML cases and 0.17 ppm for MPD cases. | Continuous or categorical analysis showed no increased risk of CML or MPD with any benzene exposure metric. No significant increased risk was highlighted for those who were highly exposed. Minimal association was seen for spline analyses for MPD if exposures occurred 2 to 20 years before diagnoses when peak exposures were considered with cumulative exposure as a continuous variable. Lack of sufficient association between MPD, CML and low exposure to benzene was observed. | Not determined. |
Koh et al. 2011 | Retrospective cohort study of 8 866 male petrochemical industry workers. Workers worked at 1 refinery and 6 petroleum chemical companies that used or manufactured benzene in Korea between 1960 and 2007. | No true estimation of job exposure levels was conducted in this study. The authors stated the production of a job-exposure matrix was inconceivable due to the lack of data on work environment exposure and old job history. | SMR for leukemia (2.12, 95% CI: 0.69–4.96) and lymphohematopoietic cancers (1.72; 95% CI: 0.69–3.54) was increased in comparison to the general population. Workers were divided by their job title into 4 groups: production workers had an SMR for leukemia of 2.77 (95% CI 0.85–7.09) and an SMR of 2 for LH (95% CI: 0.65–4.66). Leukemic cancer SIR increased (1.40; 95% CI: 0.29–4.08) for manufacturing industry workers in comparison to the general population that had increased SIRs for NHL (0.70; 95% CI: 0.008–2.52) and LH cancers (0.92; 95% CI: 0.30–2.14). SIRs calculated for all job title positions showed no significant increased risks for leukemia or LH cancers, respectively, (2.24; 95% CI: 0.46–6.54), (1.47; 95% CI: 0.48–3.44). | Not determined. |
Koh et al. 2014 | Retrospective cohort study of 14 698 male petrochemical workers at a regional petrochemical plant in Korea during the years 2002 to 2007. Participants were aged 20 to 72. | Petroleum workers were exposed to other carcinogens besides benzene. In a previous study, the benzene monitoring study defined the estimated exposure levels of benzene. The long-term geometric mean of benzene exposure was 0.025 ppm and the short-term exposure level was 0.020 ppm. | Standardized incidence ratio of NHL was 1.83 (95% CI: 0.38–5.34) and the standardized mortality ratio was 1.24 (95% CI: 0.30–4.27). Adjustments were not made for alcohol or cigarette use. | Not determined. |
Collins et al. 2015 | Retrospective occupational cohort study of the mortality status of 2 266 workers exposed to benzene at the DOW Chemical plant in US. The study ran from 1940 to 2009. A total of 1 590 deaths were reported for a total of 94 892 person-years. | Exposure to benzene was measured from industrial hygiene reports from 1944 until plant closure. Individual employee exposure was determined by work history information and job exposure levels to determine the average daily benzene exposure. Individual average daily benzene exposures were categorized into 5 sections: less than 1 ppm (average 0.5 ppm); 1 to 2 ppm (average 1.5 ppm); 2 to 9 ppm (average 5 ppm); 10 to 24 ppm (average 15 ppm); 25 ppm or more (average 30 ppm). The average duration of exposure to benzene was 4.9 years (range, 30 days to 44.7 years). The average cumulative exposure was 35.1 ppm-years (range: 0.5 to 499.5 ppm-years). |
Average exposure to benzene and its association with leukemia mortality showed that all leukemias have a standardized mortality rate (SMR) of 1.21 (95% CI: 0.74–1.97). 20 leukemia deaths occurred. For ANLL the SMR was = 1.04 (95% CI: 0.34–2.44). 5 ANLL deaths occurred. No trend for cumulative exposure levels was observed. The standardized mortality ratio of NHL was 0.97 (95% CI: 0.54–1.60). For workers with more than 30 years' latency, the standard mortality ratio was 1.02 (95% CI: 0.53–1.78). | Not determined. |
Villeneuve et al. 2014 | Case-control study of 445 cases of lung cancers and 948 controls. Additional co-pollutants measured include NO 2 and an undefined list of VOCs. | Benzene exposure concentrations were measured in outdoor air at 50 locations in Toronto from a study in 2006. A model was used to estimate exposures at residences for 3 different time periods. The time periods included: 1) exposure at time of interview or diagnosis; 2) exposure 10 years prior to diagnosis or interview; and 3) time-weighted average across all residences for the 10 years up to diagnosis or interview. The median benzene concentration was 0.65 μg/m3 in cases and 0.62 to 0.64 μg/m3 in controls. | The authors observed that an IQR (0.15 μg/m3) increase in time-weighted average benzene exposure across past residences was associated with an OR of 1.84 (95% CI, 1.26–2.68) after model adjustment for age, sex, smoking, second-hand smoke, and other confounders. Other co-pollutants such as NO 2 and total hydrocarbons showed significant relationships with lung cancer diagnoses. No other analysis was done to distinguish the effects of benzene from those of other co-pollutants. | LOAEC for increased risk of lung cancer = 0.15 µg/m3 as an outdoor air residential benzene. |
Filippini et al. 2019 | Systematic review and dose-response meta-analysis of the relationship between outdoor air pollution and childhood leukemia | Benzene exposure was evaluated by proxy exposures: traffic-related metrics near the child's residence (number of vehicles on the closest roads, road density, and distance from major roads) | The study evaluated risk of childhood leukemia for various traffic-related metrics near the child's residence (number of vehicles in the closest roads, road density, and distance from major roads) separate from an evaluation to be benzene and certain other pollutants (NO2, 1,3-butadiene, and PM2.5). When the highest category of exposure was compared to the lowest, benzene was found to be associated with an increased risk of childhood leukemia and particularly AML in children under 6 years of age (RR of 1.39 for all leukemia; RR of 3.21 for AML; RR of 1.19 for acute lymphoblastic leukemia). A higher pooled risk estimate for benzene exposure was observed in preschoolers compared to school-aged children. There was little association between childhood leukemia risk and traffic-related metrics near the child's residence. The authors concluded that the study supports an association between benzene and childhood leukemia risk and further noted that there appears to be no threshold for this effect. They also indicated that there could be a role for other traffic-related pollutants including unmeasured pollutants. | Not determined. |
Gong et al. 2019 | Meta-analysis of traffic-related pollution and risk of childhood leukemia including 21 case-control studies. | Benzene exposure was characterized as ever/never, or as low, medium or high in different analyses. | The risk of childhood leukemia associated with moderate exposure to benzene was (OR: 1.04, 95% CI: 0.71–1.37); with moderate exposure to NO 2 was (OR: 1.02, 95% CI: 0.93–1.10); and with overall traffic density was (OR: 1.01, 95% CI: 0.98–1.04). No significant association was found between benzene exposure and childhood leukemia risk. | Not determined. |
Filippini et al. 2015 | Meta-analysis of benzene and childhood leukemia via outdoor air and exposure associated with traffic density, traffic-related pollution or gas station proximity | Various methods to estimate benzene in outdoor air including proxy exposures, measurement and models. | ORs for increased leukemia risk in the highest benzene exposure category was 1.64 (95% CI: 0.91–2.95) for total leukemia, 1.09 (95% CI: 0.67–1.77) for ALL and 2.28 (95% CI: 1.09–4.75) for AML. ORs tended to be higher in European vs. American studies. Elevated ORs were also found for NO 2 (but not as high as for benzene). | Not determined. |
Vinceti et al. 2012 | Case-control study of children aged 14 and below diagnosed with leukemia. Study included 83 cases of leukemia; 64 of acute lymphoblastic leukemia (ALL); 19 cases of AML. In all, 332 controls were matched. PM 10 was also evaluated in this study. | Benzene exposure was measured from outdoor concentrations modelled from motorized traffic near the residence of each participant at the date of diagnosis. Benzene concentrations were estimated in the quartiles as averages and maximum hourly concentrations. For average concentrations, the quartiles were: <0.10 μg/m3; 0.10-< 0.25 μg/m3; 0.25-<0.50 μg/m3; and >0.5 μg/m3. For maximum hourly concentrations, the quartiles were as follows: < 02 μg/m3; 2 <4 μg/m3; 4 <6 μg/m3; and >6 μg/m3. | For an increase in 1μg/m3 of average outdoor benzene exposure, OR was significantly greater than 1.0 for AML cases in children <5 years of age (adjusted OR = 5.46 (95% CI: 1.12–26.51)). Some ORs were greater than 1 but not at a level of significance for AML in all ages for an increase of 1μg/m3 as an average concentration. Children 5 and older: the ORs were 0.43 for an increase of 1μg/m3 as an average concentration. Comparison between the quartiles showed that benzene did have a dose-response relationship, the only significant relationship was observed for the highest quartile, OR of 3.3. The maximum hourly concentrations did not seem to have as great of an effect as average concentrations. ORs for benzene described above were adjusted for exposure to PM10. | LOAEC for 92% increased risk of AML was an average increase of 1 μg/m3 as an outdoor air residential benzene concentration. |
Janitz et al. 2017 | Case-control study of 307 childhood cases of leukemia with 1 013 controls matched to week of birth. | Outdoor benzene concentration was modelled at birth residence and estimated by using the US government's measurements. Concentrations were organized in quartiles: 0.11 to <0.39 μg/m3; 0.39 to <0.67 μg/m3; 0.67 to <0.91 μg/m3; and 0.91 to 2.03 μg/m3. | No significant differences were found from overall benzene exposure concentrations between the cases and controls. Some trends indicated elevated ORs for higher quartile exposures compared to the lowest quartile. AML had a stronger association than acute lymphoid leukemia. Adjustments were made for maternal education, urbanization, ethnicity, age at diagnosis, gender, birth order, exposure to electromagnetic fields and maternal specific variables concerning education, age and smoking during pregnancy. No other pollutants were evaluated. | Not determined. |
Appendix C: Toxicological studies
Study | Species, sex, number | Exposure | Results | NOAEC/ LOAEC |
---|---|---|---|---|
Weaver et al. 2007 | Male (outbred) Sprague-Dawley rats. 10 per group. |
0 or 300 ppm benzene for 23 hours for 7 consecutive days | Cells of the parenchymal lung tissue underwent apoptosis after exposure to benzene. Indications of apoptosis were change of chromatin condensation, nuclei segmentation, and cell shrinkage. Epithelial of large bronchioles had the highest rate of apoptosis (73.5%) followed by the terminal bronchioles (65%), respiratory bronchioles (60.8%) and alveoli (55.6%). These tissues all equally had significant increase of apoptotic activity in comparison to the controls (p ≥0.01). Increased indicators of DNA fragmentation was reported in the pulmonary tract in comparison to controls (p <0.001). Caspase 1 effects were located to the bronchiolar cells mainly in the apical epithelia and newly apoptotic cells. Significant increase of caspase-1, 2, 8, and 9 activity was observed in benzene- exposed rats' pulmonary tract cells compared to controls (p <0.001). | LOAEC = 300 ppm |
Rozen et al. 1984 | 8-week-old male C57B1 mice. 7 to 8 per group. |
0, 10.2, 30.1, 101, or 303 ppm benzene. 6 hours/day for 6 days |
At all concentrations, lymphocyte levels declined. Erythrocyte counts increased at 10 ppm, were unchanged at 30 ppm and declined at 100 ppm and 300 ppm. Femoral bone marrow B-cell lymphocyte colony-forming abilities were reduced to 30% of the control at all benzene exposure levels. Femoral B-lymphocyte counts reduced at 100 ppm and 300 ppm. Splenic B-lymphocyte colony-forming ability was impaired at 300 ppm. Splenic B-lymphocyte counts reduced at 100 ppm and 300 ppm. PHA stimulation of splenic T cells declined at concentrations above 10 ppm. Splenic T cell counts reduced at 100 ppm and 300 ppm. | LOAEC = 10.2 ppm (32.6 mg/m3) |
Chen, Guo et al. 2019 | 8-week-old male mice. Wild type or strain with hepatocyte-specific homozygous deletion of Ppp2r1a gene. 6 to 10 per group. |
0, 1 ppm (3.2 mg/m3), 10 ppm (32 mg/m3), or 100ppm (320 mg/m3) benzene by inhalation, 6 hours/day, 6 days/week for 4 weeks. During the 4 weeks, gavage exposures were 0, 1, 10 or 100 mg/kg, 6 days/week. | Wild type mice showed significant declines of lymphocytes at all levels of benzene concentrations for inhalation and oral exposures (p < 0.05). At high dosages, all parameters declined for the inhalation exposure group and oral exposure group except for platelet count (p <0.05). Mutant mice with PP2A deletion were less susceptible to the various levels of exposures. | LOAEC/L for hematotoxicity is 3.2 mg/m3 and 1 mg/kg bw/day |
Chen et al. 2016 | 6- to 7-week-old male, CD1 mice. 6 per group |
0 or 300 ppm (960 mg/m3) benzene, 6 hours/day, 5 days/week for 8 weeks. A subgroup of mice received an intraperitoneal injection of histone deacetylase (HDAC) inhibitor (trichostatin A (TSA) or a mixture of ribosome inactivating proteins MCP30) before daily benzene exposure. | Hematotoxicity was observed in benzene-treated mice with decreased hemoglobin, WBCs, and platelets compared to controls (p <0.01 to 0.05). Differences in mRNA levels and acetylation of Topoisomerase IIalpha promoter levels were observed (p <0.01). HDAC inhibitor supplementation restored peripheral blood counts in mice exposed to benzene and increased expression of Topoisomerase IIalpha. | N/A |
Wathier et al. 2019 | 4- to 7-month-old male Brown Norway rats. 5 per group. |
0 or 3 000 ppm (9 600 mg/m3) benzene. Animals were exposed for 15 minutes to benzene 3 000 ppm (9,600 mg/m3), and then 20 minutes of fresh air, then 15 more minutes of exposure to benzene. | Exposure to benzene significantly increased MER (middle-ear reflex) amplitude (p < 0.001). In comparison to all the other solvents tested, benzene had the biggest effect on MER. Benzene was not found to be cochlear toxic as there were no histopathological changes in comparison to the effects of other solvents. | LOAEC = 9 600 mg/m3 |
Abplanalp et al. 2017 | 10-week-old male C57BL/6 mice. 10 per group. |
0 or 50 ppm (160 mg/m3) benzene, 6 hours/day, 5 days/week for 6 weeks. | In comparison to the controls, mice with benzene exposure showed a significant reduction in circulating angiogenic cells (Flk-1+/Sca-1+) but increased levels of low-density-lipoproteins (LDL). | LOAEC = 160 mg/m3 |
Keller and Snyder 1988 | Swiss Webster mice (Cr1:CFW(SW)Br); 2 male and 2 female per group. |
0, 5, 10 or 20 ppm benzene, 6 hours/day from gestational day 6 to 15. | At post-natal day (PND) 2, reduced numbers of circulating erythroid precursor cells (early-nucleated red cells or basophilic normoblasts), with a dose-response pattern was reported at all concentrations of benzene. Exposure to 20 ppm benzene exhibited increased numbers of hepatic hematopoietic blast cells (blasts, dividing granulocytes) and non-dividing granulopoietic precursor cells (differentiated to metamyelocyte stage) accompanied by decreased numbers of late erythropoietic precursor cells (polychromatic normoblasts and their nucleated progeny). At PND 42 (6-wks) and 20 ppm, a similar pattern of enhanced granulopoeisis was observed, as well as elevated numbers of splenic hematopoietic blast cells and granulopoietic precursor cells accompanied by decreased numbers of marrow erythropoietic precursor cells. |
LOAEC = 5.1 ppm (16.3 mg/m3) |
Study | Species, sex, number | Exposure | Results | NOAEC/ LOAEC |
---|---|---|---|---|
French et al. 2015 | Randomized clinical trial of diverse outbred male mice, with 75 mice per group | Benzene exposure concentrations at 0, 1, 10 or 100 ppm. Trial ran for 28 days and inhalation exposure was for 6 hours a day, 5 days a week. Two independent cohorts. | Dose-dependent increase for benzene- induced chromosomal damage. The BMCL was estimated to be 0.205 ppm benzene. Locus on chromosome 10 (31.87 MB) contained a pair of overexpressed sulfotransferases that were inversely correlated with genotoxicity. Data for MN-RET (micronuclei frequency in reticulocytes) and MN-ERC (micronuclei frequency in mature erythrocytes) were similar. In peripheral blood at 100 ppm, there was a significant difference in MN-RET compared to the control 573% increase. Bone marrow showed a 1-unit increase in MN-RET/1000 from 0-1 ppm and 1-10 ppm, 9 unit increases from 10-100 ppm. The identified BMCL10 was 1.52 ppm (significant lack of fit) and 0.205 when the highest dose was removed. Modelling could not be performed on MN-ERC results due to high values in the control group. Estimated values from published B6C3F1 was BMCL 10 = 3.66 ppm. | BMCL 10 0.205 ppm (MN-RET) |
Bird et al. 2010 | Randomized clinical trial of 7-week-old male, CD-1 mice. Mice were in groups of 10 to 12. | Mice in inhalation chambers (1 m3) were exposed to benzene and/or toluene for 6 hours per day, either intermittently (8 days of exposures over 15 days) or continuously (daily for 8 consecutive days). In the intermittent exposure part of the study, benzene concentrations were 0, 50 ppm (160 mg/m3) or 150 ppm (480 mg/m3). Continuous benzene exposure concentrations were 0 or 50 ppm (160 mg/m3). Toluene exposure was also facilitated in concentrations ranging from 0, 50 and 100 ppm. In the continuous exposure part of the study, some animals were exposed to buthionine sulfoximine (BSO) to deplete glutathione (GSH). | Continuous exposure to benzene concentrations of 160 mg/m3 for 8 consecutive days or 8 days staggered over 15 days significantly increased MN (micronuclei) frequency (p <0.05). No comparison was done in the study, but it seems the 8 consecutive day group had double the MN frequency of the 8 intermittent day group. In the continuous component, GSH and GSSG (whole blood) glutathione/ oxidized glutathione were not significantly changed following exposure to 160 mg/m3. During continuous exposure, some urinary metabolites significantly increased at 160 mg/m3 including phenol, trans, trans-muconic acid, hydroquinone, trihydroxybenzene and S-phenylmercapturic acid. Co-exposure with toluene doubled MN frequency. Pretreatment with BSO decreased GSH levels but also decreased MN frequency. The authors indicated that this is consistent with a benzene GSH conjugate being responsible for genotoxicity. | LOAEC for increased MN frequency: 160 mg/m3 |
Abplanalp et al. 2019 | Randomized clinical trial including 10-week-old wild type C57BL/6, male mice. Mice were in groups of 10 to 34. | In inhalation chambers, benzene was exposed to mice at a concentration of 0 (HEPA filtered air) or 10 ppm (32 mg/m3) for 6 hours per day, 5 days per week for 2 weeks; or 50 ppm (160 mg/m3) for 6 hours per day, 5 days per week for 2 or 6 weeks. Some animals were treated with superoxide dismutase mimetic (TEMPOL) to determine if benzene effects could be reversed. | Mice exposed to 32 mg/m3 for 2 weeks showed no significant effects. Mice exposed to 160 mg/m3 showed significant biochemical indicators for glucose intolerance for 2 or 6 weeks (p <0.05). Mice exposed to 160 mg/m3 showed signs of insulated-stimulated Akt phosphorylation in liver and skeletal muscle (p <0.05); oxidative stress and inflammation of liver and muscle tissue (p <0.05); and supressed phosphorylation (p <0.05). TEMPOL treatment reversed some of the benzene effects on oxidative stress, Nf-jb phosphorylation, Socs1 expression, Irs-2 tyrosine phosphorylation, and systemic glucose intolerance. | LOAEC 160 mg/m3 |
Appendix D: Other inhalation reference exposure levels
D1. Short-term inhalation reference exposure levels
The ATSDR (2007) developed an acute Minimal Risk Level (MRL) of 29 μg/m3 for 14 days or less based on a LOAEC of 32.6 mg/m3 (10.2 ppm) for reduced lymphocytic proliferation following mitogen stimulation in mice exposed to benzene 6 hours/day for 6 days (Rozen et al. 1984). The LOAEC was adjusted for continuous exposure (6 hours to 24 hours), and uncertainty factors were applied for interspecies differences (3), human variability (10) and use of a LOAEC (10).
The ATSDR (2007) developed an intermediate MRL of 19 μg/m3 for 15 to 365 days based on an LOAEC of 32 mg/m3 (10 ppm) for delayed splenic lymphocyte response to foreign antigens in mice exposed to benzene 6 hours/day, 5 days/ week for 4 weeks (Rosenthal et al. 1987). The LOAEC was adjusted for continual exposure (6 hours to 24 hours and 5 days to 7 days), and uncertainty factors were applied for interspecies differences (3), human variability (10) and use of a LOAEC (10).
ANSES (2008) selected the same endpoint, duration adjustment and uncertainty factors as the ATSDR (2007) for the 14-day MRL and developed a short-term exposure guideline of 30 μg/m3 for 1 to 14 days (difference due to rounding).
The California OEHHA (2014) developed a reference exposure level (REL) of 27 μg/m3 for a 1-hour exposure based on a LOAEC of 16 mg/m3 (5 ppm) for decreased early nucleated red cell counts in offspring of female mice exposed to benzene for 6 hours/day on days 6 to 15 of gestation (Keller and Snyder, 1988). Uncertainty factors were applied for use of a LOEAC (√10), interspecies differences (2*√10) and human variability (10*√10).
The California OEHHA (2014) developed an REL of 3 μg/m3 for an 8-hour exposure, based on its chronic REL. See Section D2 for details.
The TCEQ (2015) developed an effects screening level (ESL) of 170 μg/m3 for a 1-hour exposure based on a LOAEC of 10.2 ppm for depressed peripheral lymphocytes and depressed mitogen-induced blastogenesis of femoral B lymphocytes in mice exposed to benzene for 6 hours/day for 6 days (Rozen et al. 1984). The LOAEC was adjusted to a 1-hour concentration [(LOAEC)3 x (6 hours/1 hour)] 1/3 and uncertainty factors were applied for interspecies differences (3), human variability (10) and use of a LOAEC (3), resulting in a reference value (ReV) of 580 μg/m3. A target hazard quotient of 0.3 was applied to the ReV, resulting in the ESL.
Organization | Reference exposure level (μg/m3) | Exposure time | Health effect | Key reference(s) |
---|---|---|---|---|
ATSDR (2007) | 29 | 14 days or less | Hematotoxicity | Rozen et al. 1984 |
ATSDR (2007) | 19 | 15 to 365 days | Immunotoxicity | Rosenthal et al. 1987 |
ANSES (2008) | 30 | 1 to 14 days | Hematotoxicity | ATSDR 2000; Rozen et al.1984 |
OEHHA (2014) | 27 | 1 hour | Developmental hematotoxicity | Keller and Snyder 1988 |
OEHHA (2014) | 3 | 8 hours | Hematotoxicity | Lan et al. 2004 |
TCEQ (2015) | 170 | 1 hour | Hematotoxicity | Rozen et al. 1984 |
D2. Long-term inhalation reference exposure levels for non-cancer effects
The US EPA (2002) developed a chronic reference exposure value of 30 μg/m3 based on a BMCLSD(8-hour TWA) of 23.0 mg/m3 (7.2 ppm) for decreased lymphocyte counts in workers occupationally exposed to benzene (Rothman et al. 1996). The BMCL was adjusted for continuous exposure (10 m3 to 20 m3 and 5 days to 7 days), and uncertainty factors were applied for effect-level extrapolation (3), human variability (10), subchronic-to-chronic extrapolation (3), and database deficiencies (3).
The ATSDR (2007) developed a chronic MRL of 10 μg/m3 based on a BMCL0.25SD of 320 μg/m3 (100 ppb) for decreased peripheral lymphocyte counts in workers occupationally exposed to benzene (Lan et al. 2004). The BMCL0.25SD was adjusted for continuous exposure (8 hours to 24 hours and 6 days to 7 days), and an uncertainty factor of 10 was applied for human variability.
ANSES (2008) selected the same endpoint, duration adjustment and uncertainty factors as the ATSDR (2007) assessment, and developed a long-term exposure guideline value of 10 μg/m3.
The California OEHHA (2014) developed a chronic REL of 3 μg/m3 based on a BMCL0.5SDof 1.523 mg/m3 (0.476 ppm) for decreased peripheral blood cell counts in workers occupationally exposed to benzene (Lan et al. 2004). The BMCL 0.5SD was adjusted for continuous exposure (10 m3 to 20 m3 and 6 days to 7 days), and uncertainty factors were applied for use of a LOAEC (√10) and human variability (60).
The TCEQ developed a chronic ESL of 84 μg/m3 based on a BMCL of 23.0 mg/m3 (7.2 ppm) for decreased absolute lymphocyte count in workers occupationally exposed to benzene (Rothman et al. 1996). The BMCL was adjusted to continual exposure (10 m3 to 20 m3 and 5 days to 7 days) and uncertainty factors were applied for human variability (10) and an incomplete database (3), resulting in an ReV of 280 μg/m3. A target hazard quotient of 0.3 was applied to the ReV, resulting in the ESL.
Organization | Reference exposure level (μg/m3) | Health effect | Key reference(s) |
---|---|---|---|
US EPA (2002) | 30 | Hematotoxicity | Rothman et al. 1996 |
ATSDR (2007) | 10 | Hematotoxicity | Lan et al. 2004 |
ANSES (2008) | 10 | Hematotoxicity | ATSDR 2007; Lan et al. 2004 |
OEHHA (2014) | 3 | Hematotoxicity | Lan et al. 2004 |
TCEQ (2015) | 84 | Hematotoxicity | Rothman et al. 1996 |
D3. Inhalation unit risks for carcinogenic effects
The US EPA (2000) assessed the Pliofilm cohort by selecting a range of risk estimates from maximum likelihood estimates generated based on low-dose linearity of the Pliofilm cohort data, considering all exposure data (including exposures over 400 ppm-years) and used mean cancer mortality risk. The analysis resulted in a range of risk estimates from 8.6 x 10 -5 to 2.5 x 10 -2 ppm -1 (2.6 x10 -8 to 7.8 x10-6 (μg/m3)-1). Within this range, risk estimates fall in the lower end if the dose-response curve is sublinear at low doses, and in the higher end if the dose-response curve is supralinear at low doses. The US EPA derived a final range of risk estimates, using default low-dose linear extrapolation, as they concluded there was a lack of convincing evidence to use another model. The final range of inhalation unit risk estimates is 2.2 x 10-6 to 7.8 x 10-6 (μg/m3)-1, which corresponds to a concentration of 1.3 to 4.5 μg/m3 associated with a risk level of 1 x 10-5.
The World Health Organization (WHO) used the 1994 risk calculation for the Pliofilm cohort developed by Crump (1994) to derive a risk estimate for benzene carcinogenicity. Using multiplicative risk estimates and a cumulative linear model that gave equal weight to concentration and duration of exposure, the WHO derived an inhalation unit risk of 1.4 to 2.3 x 10 -5 ppb -1 (4.4 to 7.5 x 10-6 (μg/m3)-1). The GM of the range of estimates of the excess lifetime risk of leukemia at an air concentration of 1 μg/m3 is 6 x 10–6. The concentrations of airborne benzene associated with an excess lifetime risk of 1/100 000 is 1.7 μg/m3.
The TCEQ (2015) used the linear model risk analyses of the Pliofilm cohort conducted by Crump (1994) to develop a cancer potency estimate for AMML. It concluded that the best-fitting linear model for AMML was based on using the weighted cumulative exposure as the exposure metric. At a target risk level of 10-5, the inhalation unit risk was determined to be 7.1 x 10-6 ppb -1 (2.2. x 10-6 (μg/m3)-1). The corresponding air concentration is 1.4 ppb (4.5 μg/m3).
Health Canada recommended a TRV of 1.6 x 10 -2 (mg/m3) -1 unit risk for inhalation exposure to benzene at federal contaminates sites. The TRV was a unit lifetime leukemia risk to the general population, derived from the Pliofilm and Chinese worker cohorts, based on the OEHHA risk analysis (2001) and the discussion in Health Canada (2013a). Poisson regression and linear relative risk models were used to derive an inhalation unit risk for lifetime inhalation exposures of the general population (based on the GM of upper bound estimates of leukemia risk from these studies) (Health Canada 2021b).
National Institute for Public Health and the Environment (the Netherlands, RIVM) developed an indoor guideline value for benzene cancer risk of 20 μg/m3 (at a maximum permissible risk of 1 case of cancer per 10 000 exposed individuals in a 100-year lifespan, equivalent to an inhalation unit risk of 5 x 10 -3 (mg/m3)-1, based on previous mortality rate analysis of the Pliofilm cohort conducted by the EU Working Group on Benzene in Outdoor Air (RIVM 2001, 2007). ANSES adopted an inhalation unit risk of 6 x 10 -3 (mg/m3) -1 as a guideline for indoor benzene exposure, based on the WHO model. ANSES considered the calculation of risk using both linear and non-linear models to be the most appropriate approach, as current knowledge does not allow the exact shape of the dose-response curve of benzene at low concentrations to be determined (ANSES 2008). The German Umweltbundesamt (Federal Environment Agency) recently adopted a reference value for inhalation unit risk of 4 x 10 -5 at 20 μg/m3, which corresponds to 0.1 μg/m3 at a risk level of 1x 10-6 (Umweltbundesamt 2020).
Organization | Inhalation unit risk (μg/m3) -1 |
Concentration at 1 × 10 -5 risk level | Health effect | Key reference(s) |
---|---|---|---|---|
US EPA (2000) | 2.2 x 10-6 to 7.8 x 10-6 | 1.3–4.5 μg/m3 | Leukemia | Rinsky et al. 1981, 1987; Paustenbach et al. 1993; Crump and Allen 1984; Crump et al. 1994; US EPA 1998 |
WHO (2000) | 6 x 10-6 | 1.7 μg/m3 | Leukemia | Crump et al. 1994 |
TCEQ (2015) | 2.2 x 10-6 | 4.5 μg/m3 | AMML | Crump et al. 1994 |
Health Canada (Contaminated Sites Division; 2021) | 1.6 x 10 -5 | 0.6 μg/m3 | Leukemia | Health Canada 2013; OEHHA 2001 |
ANSES (2008) | 6 x 10-6 | 2 μg/m3 | Leukemia | WHO 2000; Crump et al. 1994 |
RIVM (2007) | 5 x 10-6 | 2 μg/m3 | Cancer | RIVM 2001 |
Umweltbundesamt (2020) | 1 x 10 -5 | 1* μg/m3 | Leukemia | AGS 2012 |
*The provisional guideline value is 4.5 μg/m3 (Umweltbundesamt 2020). |
Footnotes
- Footnote 1
-
The terms "women" and "men" are used in the original study report. It is not clear from the report whether sex or gender were considered in the analysis.
Page details
- Date modified: